Comodulation of NO-Dependent Vasodilation by Erythroid Band 3 and Hemoglobin: A GP.Mur Athlete Study
- 1Department of Medical Research, MacKay Memorial Hospital, New Taipei City, Taiwan
- 2MacKay Junior College of Medicine, Nursing, and Management, New Taipei City, Taiwan
- 3Institute of Biomedical Sciences, Mackay Medical College, New Taipei City, Taiwan
- 4Department of Critical Care Medicine, MacKay Memorial Hospital, New Taipei City, Taiwan
- 5Department of Physical Education, University of Taipei, Taipei, Taiwan
- 6Department of Chemical Engineering, National Chung-Cheng University, Chia-Yi, Taiwan
- 7Division of Gastroenterology, Department of Internal Medicine, MacKay Memorial Hospital, Taipei, Taiwan
- 8Department of Medicine, MacKay Medical College, New Taipei City, Taiwan
- 9Department of Exercise and Health Sciences, University of Taipei, Taipei, Taiwan
- 10Division of Cardiology, Departments of Internal Medicine, MacKay Memorial Hospital, Taipei, Taiwan
GP.Mur, a red blood cell (RBC) hybrid protein encoded by glycophorin B-A-B, increases expression of erythroid band 3 (Anion Exchanger-1, SLC4A1). GP.Mur is extremely rare but has a prevalence of 1–10% in regions of Southeast Asia. We unexpectedly found slightly higher blood pressure (BP) among healthy Taiwanese adults with GP.Mur. Since band 3 has been suggested to interact with hemoglobin (Hb) to modulate nitric oxide (NO)-dependent hypoxic vasodilation during the respiratory cycle, we hypothesized that GP.Mur red cells could exert differentiable effects on vascular tone. Here we recruited GP.Mur-positive and GP.Mur-negative elite male college athletes, as well as age-matched, GP.Mur-negative non-athletes, for NO-dependent flow-mediated dilation (FMD) and NO-independent dilation (NID). The subjects were also tested for plasma nitrite and nitrate before and after arterial occlusion in FMD. GP.Mur+ and non-GP.Mur athletes exhibited similar heart rates and blood pressure, but GP.Mur+ athletes showed significantly lower FMD (4.8 ± 2.4%) than non-GP.Mur athletes (6.5 ± 2.1%). NO-independent vasodilation was not affected by GP.Mur. As Hb controls intravascular NO bioavailability, we examined the effect of Hb on limiting FMD and found it to be significantly stronger in GP.Mur+ subjects. Biochemically, plasma nitrite levels were directly proportional to individual band 3 expression on the red cell membrane. The increase of plasma nitrite triggered by arterial occlusion also showed small dependency on band 3 levels in non-GP.Mur subjects. By the GP.Mur comparative study, we unveiled comodulation of NO-dependent vasodilation by band 3 and Hb, and verified the long-pending role of erythroid band 3 in this process.
Introduction
Hemoglobin (Hb) modulates vascular tone by controlling nitric oxide bioavailability in the blood stream. The chemical reaction between Hb and NO metabolites is coupled with the dynamics of O2 saturation and desaturation of Hb (1–5). While intraerythrocytic Hb is considered the most powerful NO scavenger in human body, its effect on NO-associated vasodilation is limited by the RBC membrane (6). Hb-mediated NO bioavailability has been proposed to require band 3, the main anion transporter on RBCs. The interaction between band 3 and deoxygenated Hb in the submembranous domain has been suggested to assist the transport of NO metabolites (e.g., nitrite and SNO) across the red cell membrane by unclear means (7–10). Currently, the role of band 3 in RBC-dependent NOx processing and hypoxic vasodilation is enigmatic.
GP.Mur (also known as Miltenberger subtype III or Mi.III), is a Southeast Asian special blood type with 1–10% prevalence among Filipinos, Vietnamese, Thai, and Taiwanese, but is very rare among Caucasian (0.0098%), northern Asian like Han Chinese and Japanese (0.006%) (11–14). Biochemically, GP.Mur is a hybrid protein originated from homologous gene recombination of the highly homologous GPYB and GYPA genes (15). As GP.Mur contains a segment of glycophorin A (GPA) and GPA acts as a chaperone to band 3 (16, 17), the consequence of the expression of GP.Mur is to support increased expression of band 3 on the erythrocyte surface (18). Band 3, a Cl−/ antiporter, allows permeation across the erythrocyte membrane, which is physiologically essential for intraerythrocytic CO2/ conversion and postnatal CO2 respiration (19–21). People with GP.Mur blood type express more band 3 on RBCs and show faster CO2 respiration after a mild physical challenge (18, 22). Unexpectedly, from our previous human exercise study on Taiwanese adults aged up to 50 years old, GP.Mur+ subjects exhibit slightly higher blood pressure than the non-GP.Mur counterparts (22, 23).
The structural-functional hallmarks of GP.Mur+ red cells are enhanced band 3 expression (18) and altered band 3 complex structure with other membrane proteins on the red cell surface (21, 24–26). GP.Mur+ red cells present stronger band 3-AQP1 interaction (18, 26), contain reduced amount of RhAG and consequently altered band 3-Rh/RhAG macrocomplex organization (25, 27), and are more resilient physically in the osmolarity-fragility test (18). These presumably could affect band 3 interaction with submembranous deoxy Hb and metHb (28) and/or result in differential NOx processing, vasodilation, and blood pressure setting. To test this hypothesis, we examined NO-dependent FMD and NO-independent vasodilation primarily in a uniform cohort—Taiwanese elite college athletes who have been trained professionally for national and/or international sports competitions for approximately half of their lifetime. Together with blood measurements for band 3 and NOx changes during FMD, we identified co-modulation of FMD by erythroid band 3 and Hb.
Materials and Methods
Subject Recruitment, GP.Mur Phenotyping, and Study Design
This human study protocol had been approved by MMH-Institutional Review Board (IRB registration: 19MMHIS081e). For uniform cohorts, we primarily recruited male elite athletes from top sports universities in Taiwan, where they enrolled with their competition scores from national/international games. Before college, all of them had professional sports training and competition experiences for at least 6 years. All subjects were free from major illness. To maintain eligibility for sports competitions, they were prohibited from taking most medication. We also recruited age- and BMI-matched, healthy male non-athletes as controls. All subjects had given written informed consents before participating in this study.
GP.Mur blood type was first determined by red cell serology using anti-Mur and anti-Mia antisera, and then confirmed by PCR-sequencing, as described (29). It has long been observed in Taiwan that the prevalence of GP.Mur among elite Taiwanese athletes is much higher. We pre-screened 129 elite male college athletes and found 32 of them with GP.Mur blood type (24.8%). Among the elite athletes, 51 of them agreed to participate in this study, and 19 of them bear GP.Mur phenotype (37.3%). As for the non-athlete subjects, only one out of 20 bear GP.Mur (5%), which is similar to the occurrence frequency of GP.Mur in general Taiwanese population.
The study design was illustrated in the flow chart in Figure 1. Briefly, each subject was tested on two separate days: 1 day for ultrasound measurements of FMD and then NID; the other day for assessments of the changes of blood plasma NO metabolites during FMD (blood withdrawal mostly completed within 1 min after cuff deflation). The subjects were not allowed intake of food or caffeinated drinks at least 2 h prior to FMD tests on both days. They were asked not to practice sports at least 6 h before testing.
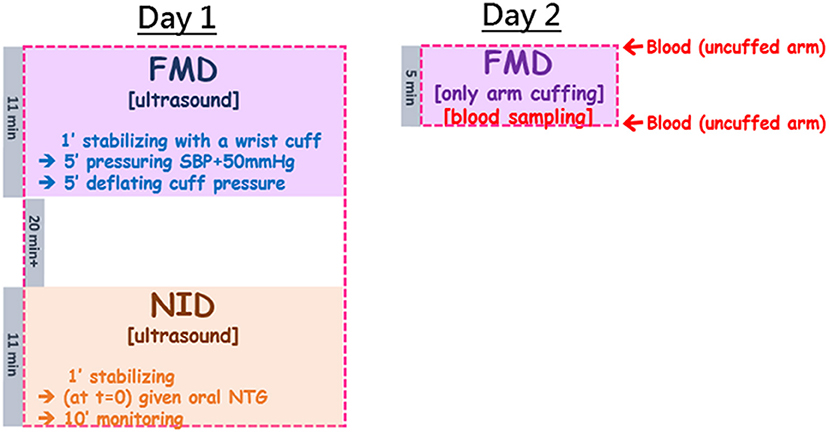
Figure 1. The study flow chart combined ultrasound measurements of FMD and NID, and blood sampling before cuffing and right upon pressure deflation which allowed assessment of plasma NOx changes by arterial occlusion during FMD. The study was arranged in two separate days: Day 1 for ultrasound-based tests; Day 2 for blood sampling before and after 5-min brachial arterial constriction. In Day 1, the subject was started with the ultrasound measurement for FMD, followed by a minimal 20-min break to allow recovery of the tested brachial artery, and then the second ultrasound measurement for NID. FMD took ~11 min (including 1 minute of stabilization, 5 min of cuff pressuring to 50 mmHg above the subject's SBP, and another 5 min of observation after wrist cuff deflation). The NID measurement also began with 1-min stabilization, followed by oral administration of NTG and then 10-min monitoring of the changes of the brachial arterial diameter. In Day 2, the setting was very similar to FMD in Day 1 (5 min of pressuring to 50 mmHg above the subject's SBP), but ultrasound was replaced by blood sampling before and after cuffing.
Ultrasound Measurements for FMD and NID
We followed the FMD and NID protocols provided by the Hitachi-Aloka eTRACKING (echo-tracking) system (30). The Aloka eTRACKING program was installed in the Hitachi Arietta 60 (Ar60) ultrasound system equipped with a 12–2 MHz transducer (L441).
Before ultrasound examination, each subject was allowed to calm down in bed in a dark, quiet examination room, and his left wrist was wrapped with a pneumatic cuff. The L441 transducer locked in a probe holder was placed about 5–10 cm above his left elbow to image his left brachial artery. Under the FMD mode of eTRACKING, a selected region of his brachial artery was first focused and diameter changes in the focused brachial artery were recorded over time. The FMD recording began with 1-min baseline (stabilization), 5-min inflation of the wrist pressure to 50 mmHg above his systolic blood pressure (SBP), followed by deflation and 5 more minutes of monitoring the changes of the brachial arterial diameter. The peak of reactive hyperemia generally appeared within 30–90 s post-arterial occlusion, and the brachial arterial diameter generally returned to its baseline level within 2 min (see Figure 2A).
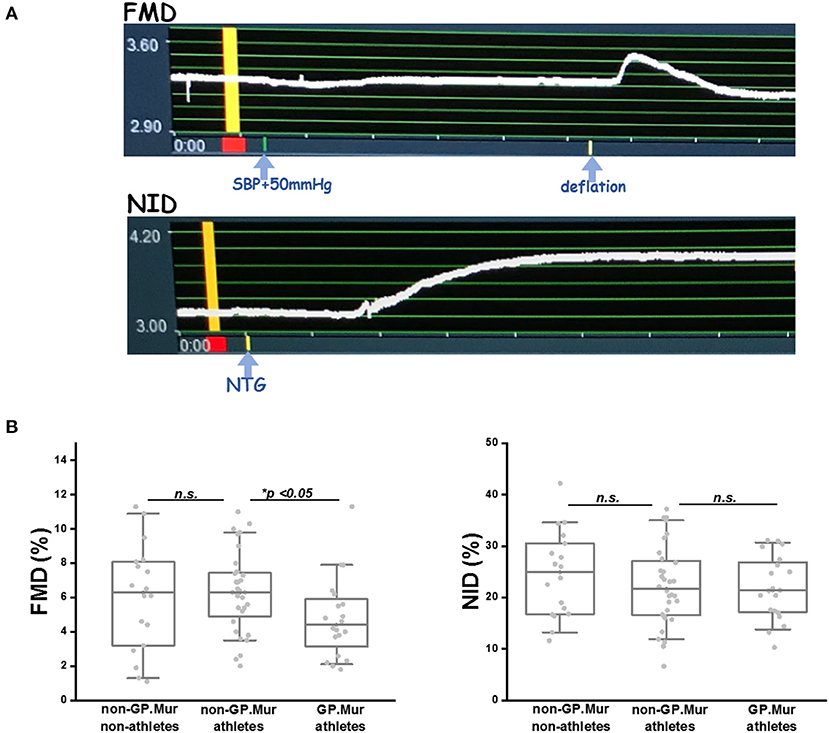
Figure 2. FMD was significantly lowered in GP.Mur+ male elite athletes than GP.Mur-negative athletes or nonathletes, whereas NID was not affected by GP.Mur expression. (A) TOP: An example of FMD captured by the eTRACKING program of Hitachi-Aloka ARIETTA 60. The diameter of the region tracked is shown at the y-axis (unit: mm). The recorded time is shown at the x-axis (unit: minute). The yellow bar indicates the baseline of the vessel diameter that was to be compared with the maximally dilated diameter of the same region for %FMD or %NID. BOTTOM: An example of NO-independent vasodilation. (B) FMD was compared among the three groups of subjects—GP.Mur athletes, non-GP.Mur athletes, and non-athletes. Each dot represented the datum of a subject. The box covered data ranging 25–75%; the line inside a box indicated the median value. Data between the paired groups were compared by two-sample t-test (*p < 0.05). RIGHT: Comparison of NID (n. s., not significant).
After FMD, the subject was given a 30-min break and then stabilized again in bed for ultrasound examination of NID on a similar region of the brachial artery as for FMD. NO-independent dilation recording by eTRACKING also began with 1-min baseline. The subject was then given 0.6 mg sublingual nitroglycerin (Nitrostat®, Pfizer), and his changes of the brachial arterial diameter were recorded for 10 min. These subjects generally reached maximal vasodilation by the 6th minute post-nitroglycerin. Nitroglycerin-induced hyperemia generally persisted till the end of the total 11-min recording (see Figure 2A).
Determination of Blood Plasma Nitrate and Nitrite Concentrations
To find out blood plasma NOx changes during FMD, on a separate visit the subject underwent a similar mechanical stimulus–5-min cuff inflation to 50 mmHg above his SBP on his right upper arm. Venous blood was sampled from his left median cubital vein at two timepoints: (1) before cuffing; (2) after deflation of the arm cuff. This allowed us to evaluate the consequence of regional ischemia and onset of NO-dependent vasodilation reflected by plasma NOx. Our blood sampling protocol for NOx during reactive hyperemia followed a report by Allen et al. (31), with slight modification.
Right after blood withdrawal into an ACD tube, the sample was immediately centrifuged to separate plasma from RBCs. Blood plasma was further filtered using an Amicon Ultra-4 Centrifugal unit with 10-kDa cutoff at 3,220 × g for 30 min at 4°C, and then aliquoted and stored at a −80°C freezer. Due to the short lifetime of nitrite, the handling process from blood withdrawal to sample storage in −80°C was limited to 45 min or less. Plasma NOx and were quantitated using the Nitrate/Nitrite colorimetric assay kit (Cayman Chemical, MI, USA); plasma was quantitated with the Nitrate/Nitrite fluorometric assay kit (Cayman).
Band 3 Quantitation
RBCs were hypotonically ruptured to obtain the membrane fraction (ghost). Total proteins in freshly lysed ghosts were quantified by Lowry protein assay (Bio-RAD, CA, USA), and band 3 levels measured by band 3 sandwich ELISA. The band 3 sandwich ELISA was developed in house. Briefly, anti-band 3 rat monoclonal BRAC 18 (International Blood Group Reference Laboratory [IBGRL], NHS, UK) was applied as the coating antibody (1:500 dilution) onto an ELISA plate overnight at 4°C. RBC ghost lysates were then reacted with the coated BRAC 18 for 30 min at 37°C, followed by PBST (0.05% Tween-20-supplemented PBS) washes and incubation with another anti-band 3 mouse monoclonal BRIC 170 (IBGRL; 1:500 dilution) for 2 h at 37°C. The signal of BRIC 170-sample binding was amplified by incubation with 1:5,000 HRP-conjugated, anti-mouse-specific secondary IgG (Jackson ImmunoResearch Laboratories, PA, USA); this secondary anti-mouse antibody had been immunoaffinity-purified to yield minimal cross reactivity with rat. The standard curve for absolute quantitation of band 3 in the sandwich ELISA used a custom-made band 3 peptide that contains the known epitope sequence of BRIC 170 (AA 368-382); the custom-made peptide sequence is NGGPDDPLQQTGQLFGGLVR (AA 365-384 of human band 3 ([NP_000333.1]). One's band 3 level relative to all RBC membrane proteins in his sample (w/w) was obtained by dividing the band 3 concentration by the ghost protein concentration and expressed in percentile (%).
Results
To probe into the potential impacts of GP.Mur/band 3 on NO-dependent reactive hyperemia in a uniform cohort, this study recruited primarily male elite college athletes from top-ranking Taiwan sports universities where the prevalence of GP.Mur is much higher than the general Taiwanese population. The athlete subjects all have similar age, lifestyle, and enrolled in rigorous sports training programs aiming for national/international competitions for approximately half of their lifetimes (Table 1). They were divided into GP.Mur-positive (19/70) and GP.Mur- negative athlete groups (32/70). For comparison, twenty age- and BMI-matched non-athlete subjects were also recruited (only 1/20 GP.Mur+). Compared to the non-GP.Mur non-athletes (19/70), both groups of athletes showed similarly lower heart rates and slightly higher systolic blood pressuer (Table 1).
Lower FMD in GP.Mur Subjects
Compared to GP.Mur-negative athletes, FMD measured by ultrasound e-TRACKER was significantly lowered in GP.Mur+ athletes (4.6 ± 2.3% [GP.Mur+ athletes] vs. 6.3 ± 2.4% [non-GP.Mur athletes], *p < 0.05), despite that their blood pressure profiles were not different (Figure 1, Tables 1, 2). Both GP.Mur+ and non-GP.Mur athlete groups exhibited similar degrees of NO-independent vasodilation triggered by nitroglycerin (Figure 2). The similar NID responses between GP.Mur+ and non-GP.Mur hint similar maximal functional capacities in their eNOS and smooth muscle cells (SMCs) (32). As for the GP.Mur-negative non-athletes, their FMD or NID were indistinguishable from either athlete groups (Figure 2, Table 2).
FMD Limited More by Hb in GP.Mur+ Than in Non-GP.Mur Subjects
In a study on hypertensive patients with high-altitude erythropoiesis, FMD in these patients was inversely correlated with their abnormally high Hb (>21 g/dL) and excessive number of circulating RBC cells (33). Here, our subjects were healthy young students with Hb and RBC count in the normal ranges. Their Hb and RBC count were not different because of GP.Mur (Table 1). But only in GP.Mur+ subjects was FMD significantly inversely correlated with Hb (Figure 3A: thick solid line). This trend would not be obvious in most non-GP.Mur people with normal Hb, as shown with the poor fitting in Figure 3A (33). If comparing the Pearson's correlation r between Hb and FMD, the correlation in GP.Mur was 4-fold that in non-GP.Mur (−0.53 [GP.Mur+] vs. −0.14 [non-GP.Mur]) (Figure 3A). From the data, Hb in GP.Mur+ RBCs was more effective in scavenging NO, and this likely involved distinct components in the GP.Mur red cell membrane. In contrast, the correlation between Hb and NID was poor for all three groups (p > 0.05), indicating that NID was not associated with Hb (Figure 3A, right). These results confirm the central role of Hb in NO-dependent vasodilation.
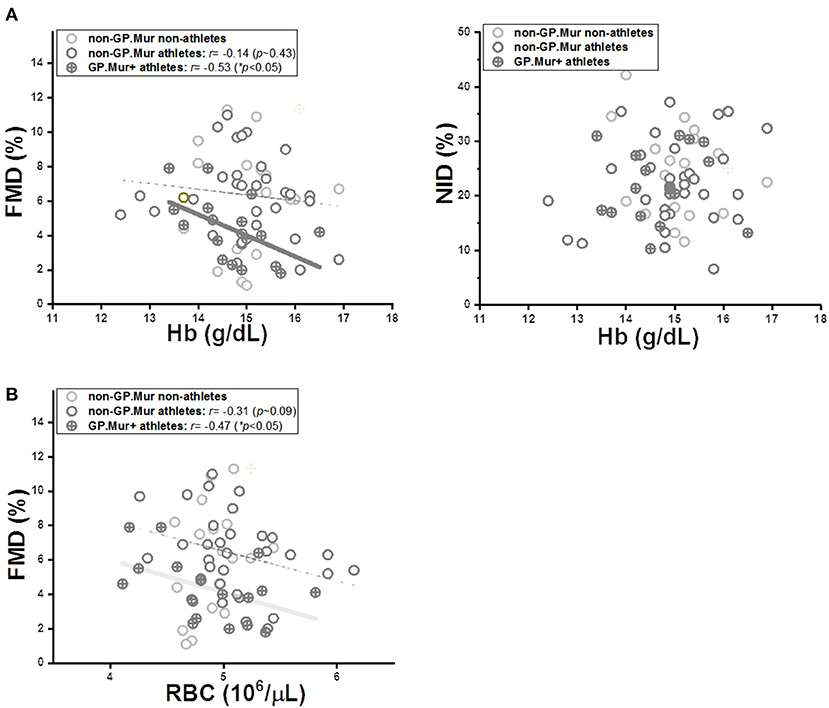
Figure 3. The limiting effect of intraerythrocytic Hb on FMD was more significant in GP.Mur+ athletes (cross circular symbols) than in the non-GP.Mur control subjects (empty circular symbols). (A) LEFT: Linear regression analyses revealed a significant inverse correlation between Hb and FMD in GP.Mur+ athletes, which was less obvious in non-GP.Mur athlete and non-athlete subjects (GP.Mur: Pearson's r = −0.53 (*p < 0.05); non-GP.Mur: weak correlation). RIGHT: Intraerythrocytic Hb exerted no specific effects on NID for GP.Mur+ or non-GP.Mur subjects. (B) The dependency of FMD on RBC count was similar for both GP.Mur+ and non-GP.Mur groups. Solid thick lines represent significant linear fitting; thin, dotted lines represent poor fitting (p > 0.05).
On the other hand, FMD correlation with RBC count was not much different between GP.Mur and non-GP.Mur (Figure 3B: r or the slopes for GP.Mur+ athletes, −0.47; for non-GP.Mur athletes, −0.31). From their similar dependency of FMD on RBC count, their drastically different FMD sensitivity to Hb had to be primarily triggered by their differences in the RBC membrane composition (i.e., GP.Mur/band 3 protein complexes).
Blood Plasma Nitrite Levels Dynamically Correlated With Band 3 Expression
Similar to the FMD/NOx study by Allen et al. (31), we observed that occlusion of the brachial artery followed by cuff deflation decreased systemic plasma nitrate and increased systemic plasma nitrite contents (Table 2, Figure 4A). But the concentrations of plasma nitrite, nitrate, or NOx before pressure cuff or right after cuff deflation were not different between GP.Mur+ and non-GP.Mur athletes (Table 2). The increase of nitrite or the decrease of nitrate by arterial occlusion and cuff release (Δ[]cuffing & Δ[]cuffing) were also not significantly different between GP.Mur and non-GP.Mur (Table 2). The level of plasma nitrite is a balance between nitrite production and nitrite consumption. Under physiologically oxygenated conditions, most nitrite is constitutively generated in tissue from oxygenation of NO produced by eNOS (34), and is consumed by reduction into NO primarily via deoxy Hb (35). Individual eNOS levels were expected to vary, and hence individuals with high []pre−FMD generally showed high []upon deflation (Figure 4B).
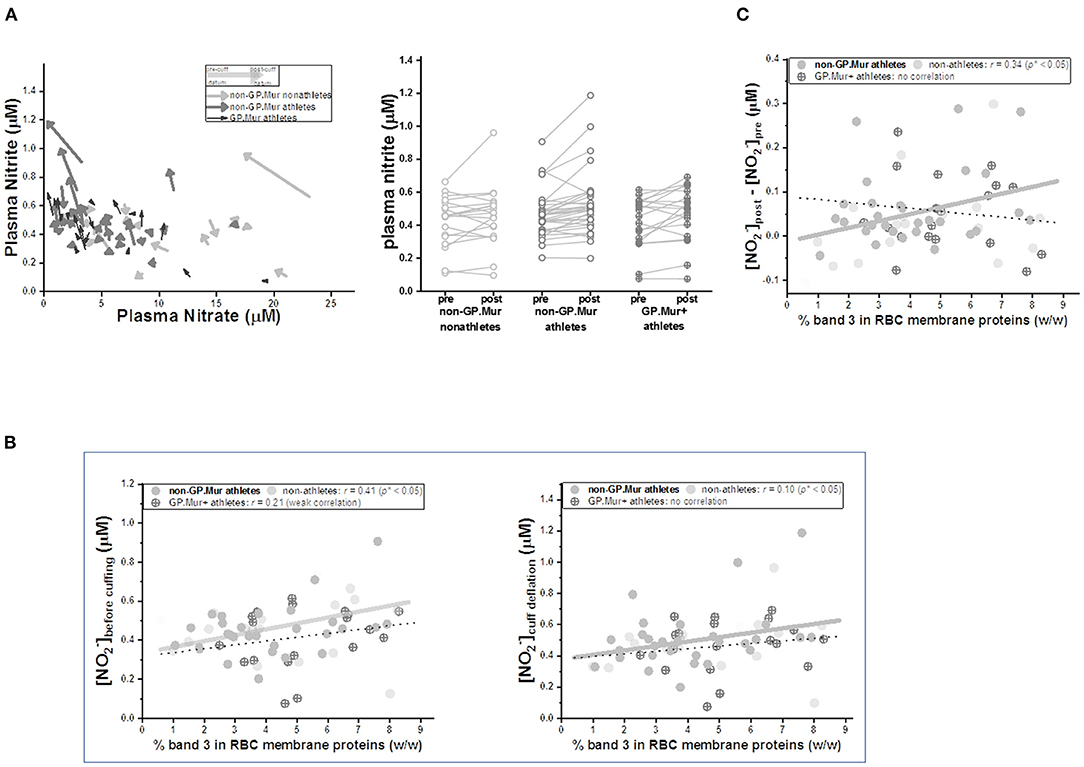
Figure 4. Arterial occlusion following cuff deflation during FMD decreased plasma nitrate and increased nitrite, the latter of which was quantitatively directly correlated with individual erythroid band 3 levels. (A) LEFT: Plasma nitrite and nitrate levels before cuffing and upon deflation were compared. Their changes (Δ[] and Δ[]) were generally inversely correlated. Each arrow represents a test subject: GP.Mur athletes in black color, non-GP.Mur athletes in dark gray color, and non-GP.Mur non-athletes in light gray color. The beginning of an arrow indicated concentrations of plasma nitrite and nitrate right before cuffing; the arrowhead indicated concentrations of plasma nitrite and nitrate upon release of the cuff pressure. RIGHT: Plasma nitrite generally increased upon cuff deflation following 5-min arterial occlusion. Each line connected the nitrite concentration before arm cuffing and the concentration upon pressure deflation for an individual subject. (B) The concentrations of blood plasma nitrite were directly proportional to individual band 3 levels. The thick gray line indicated significant linear fitting for non-GP.Mur (*p < 0.05); the thin dashed line indicated poor linear fitting for GP.Mur data (n.s.). LEFT: the concentration of plasma nitrite ([]) before cuffing. RIGHT: [] upon cuff deflation following 5-min occlusion of the brachial artery. (C) For all non-GP.Mur subjects (athletes and non-athletes), their changes of blood nitrite concentrations by arterial occlusion during FMD (Δ[]cuffing = []pre−cuff - []upon deflation) was directly correlated with erythroid band 3 levels (*p < 0.05). This direct relation between band 3 and (Δ[]cuffing) was not apparent in GP.Mur+ subjects.
Band 3 complexes have been suggested to assist Hb in NO-dependent hypoxic vasodilation, either as a nitrite transporter (7) or/and as a mediator for permeation of NOx/SNO metabolites across the red cell membrane (8, 9). Band 3 expression was slightly higher in people with GP.Mur (Table 2) (18, 22, 36). We thus explored the possible involvement of band 3 in NOx changes during FMD. The concentrations of systemic plasma nitrite before cuff pressing and after deflation were both directly correlated to band 3 expression levels on the RBC membrane (Figure 4B). The correlation between band 3 and systemic nitrite was better before than after regional arterial occlusion (Figure 4B: larger Pearson's correlation r before cuffing [left] than after cuff deflation [right]). Notably, for GP.Mur+ athletes, their band 3-nitrite correlation was much smaller and diminished after cuff deflation. We calculated the extent of systemic nitrite changes induced by this regional hypoxia ([]post - []pre, or Δ[]cuffing), which was significantly correlated with erythroid band 3 levels but only in non-GP.Mur subjects (Figure 4C). This discrepancy suggests different impacts of GP.Mur+ and non-GP.Mur membrane structure on NOx metabolism, as seen in Figure 3A.
Discussion
Intraerythrocytic Hb is the major NO scavenger inside human body, but deoxy Hb as a nitrite reductase also generates NO in low O2 during the respiratory cycle. The sources of NO for maintaining the vascular tone mainly come from constitutive conversion of L-Arginine by endothelial NO synthase (eNOS) and from reduction of NOx by heme reductase like deoxy Hb. NO production by deoxy Hb complements NO production by eNOS, which takes place preferentially in high O2. This may explain, at least in part, why eNOS expression alone cannot fully reflect NO bioavailability or FMD (37–39).
Upon deflation of the cuff pressure in FMD, we observed decrease of plasma nitrate and increase of plasma nitrite for both GP.Mur and non-GP.Mur groups (Table 2, Figure 4A). The reaction of NO in oxygenated RBCs mainly forms nitrate; the reaction of NO in oxygenated tissues mainly forms nitrite. Thus, decreasing nitrate suggests lower NO and a need to increase NO/nitrite bioavailability from both tissues and RBCs. Nitrite synthesis was accelerated to increase NO bioavailability.
This study probed into the effects of GP.Mur/band 3 on NO-dependent vasodilation. Our age-matched, young elite athletes all had similar blood pressure, but GP.Mur+ athletes had already exhibited lower FMD than non-GP.Mur counterparts (Figure 2). Their Hb levels were all within the normal range, but Hb in GP.Mur+ RBCs was more effective in limiting NO-dependent vasodilation (Figures 2, 3). This suggests that GP.Mur/band 3-associated structural features could further facilitate NO scavenge by intraerythrocytic Hb (Figure 3A). Band 3 protein complexes differ between GP.Mur and non-GP.Mur in two aspects: (1) there are more band 3 molecules on the GP.Mur+ RBCs; (2) GP.Mur+ band 3 complexes are structurally distinguishable from non-GP.Mur (18, 25–27). In particular, AQP1 and band 3 interact more strongly in GP.Mur+ than in non-GP.Mur RBCs (26). The water channel AQP1 is also a NO gas channel (40–42). Thus, we hypothesize that Hb in GP.Mur+ RBCs was more effective in scavenging NO for the two reasons: (1) more band 3 on the GP.Mur+ membrane could attract more Hb to the inner leaflet of the cell membrane; (2) their stronger AQP1-band 3 interaction could channel NO influx via AQP1 to band 3-bound Hb (Figure 5A). Though Hb was not different between GP.Mur+ and non-GP.Mur groups (Table 1), more Hb distributed on the inner leaflet would result in more efficient NO scavenge. Since NO gas diffuses into erythrocytes extremely rapidly (at a rate near its diffusion limit), the rate of NO scavenge by intracellular Hb is critical to NO bioavailability and NO-dependent vasodilation (as in Figure 3A).
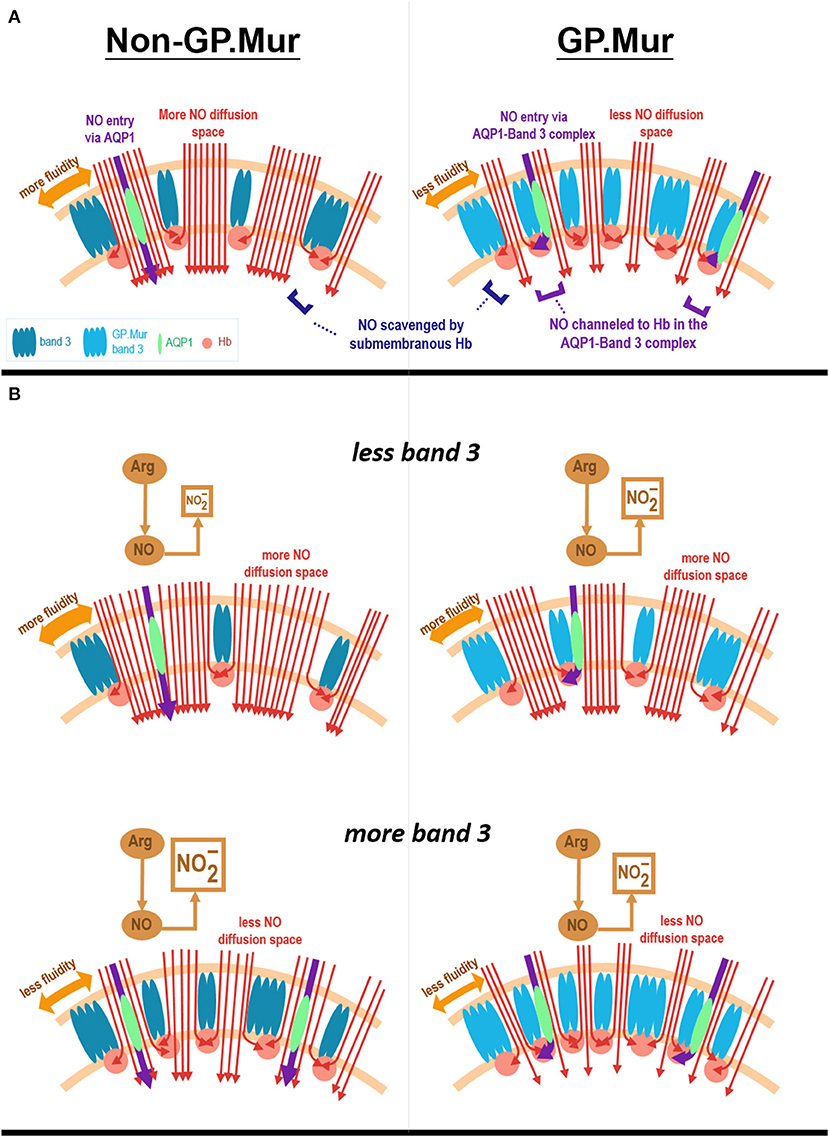
Figure 5. Hypothesis. (A) A model depicts how Hb in GP.Mur+ RBCs could be more effective in scavenging NO than Hb in non-GP.Mur RBCs. GP.Mur band 3 dimers and tetramers differ from non-GP.Mur in higher expression and distinct structural organization (labeled with different shades of blue color). Cytoplasmic band 3 binds Hb (red balls), preferentially less O2-saturated Hb. Because GP.Mur+ RBCs express more band 3, there is more Hb lining on the inner leaflet of the lipid bilayer. In terms of protein complex organization, AQP1 (green) is mobile in non-GP.Mur RBCs but is often associated with band 3 in GP.Mur+ RBCs. Their oligomerization on the GP.Mur+ membrane enables channeling of NO to Hb through the AQP1-band 3 complexes (shown in purple arrows). Both structural differences equip GP.Mur+ RBCs with a stronger suction for NO (red arrow lines). (B) A model depicts the relations between erythroid band 3 expression and plasma nitrite levels. Band 3 expression on the RBC membrane vary among individuals. The red cell membrane inserted with more band 3 protein tends to be less fluid and hence allows for less NO diffusion into the red cells (shown in red arrow lines). For non-GP.Mur (left panels), higher band 3 expression is associated with less NO entry and scavenge by erythrocytes; their plasma nitrite levels are thus higher. The content of plasma nitrite is complementary to the amount of NO scavenged by RBCs. For GP.Mur (right panels), NO diffusion into erythrocytes is not only affected by band 3 protein expression/membrane fluidity; GP.Mur+ RBCs also scavenge NO more efficiently (as explained in A). For GP.Mur, these two opposite effects conceivably even out and obscure the direct correlation between erythroid band 3 and plasma nitrite levels that was seen in non-GP.Mur.
We also found that erythroid band 3 levels were directly correlated with Δ[]FMD in non-GP.Mur but not in GP.Mur+ subjects (Figure 4). Band 3 likely could support permeation of across the red cell membrane, as proposed by other groups (7, 8). Band 3 transports at the rate of ~104/s. If band 3 transports at a similar rate as , this is still much slower than the rate of NO diffusion through the plasma membrane. Thus, the different correlations between band 3 levels and Δ[]FMD more likely involved different degrees of NO scavenge between GP.Mur and non-GP.Mur.
We hypothesize that for non-GP.Mur RBCs, a higher band 3 protein level shall decrease membrane fluidity and hence reduce NO diffusion into the red cells (Figure 5B, bottom). This is because the inner leaflet of the RBC membrane is mainly covered by metHb. Despite that metHb in RBCs is only 0.5–3%, it reacts with NO at a much slower rate than oxyHb or deoxyHb, and thus forms a barrier for NO diffusion into the red cells. Increase of band 3 expression in non-GP.Mur RBCs thus could reduce NO entry and scavenge; more plasma nitrite could then be observed (Figure 5B). On the other hand, the lack of the correlation between erythroid band 3 and plasma nitrite levels in GP.Mur+ RBCs suggest that factors other than membrane barriers to NO diffusion (i.e., metHb and fluidity) were involved. These other factors include more extensive lining of Hb with cytoplasmic GP.Mur band 3 in the inner leaflet, and unique channeling of NO to submembranous Hb via AQP1-band 3 complexes (Figure 5A). Because of these additional factors, higher band 3 levels in GP.Mur+ RBCs conceivably also promote NO scavenging. These effects were opposite to the decrease of NO entry in less fluid red cells (Figure 5B). These may explain why the direct correlation between plasma nitrite and band 3 levels seen in non-GP.Mur subjects was absent in GP.Mur (Figures 4, 5).
A final interesting observation from this study is the lower plasma nitrate (but not nitrite) among the elite athletes, regardless of GP.Mur (Table 2). Skeletal muscles are the dominant nitrate reservoir over other organs or blood (43). It has been reported that the nitrate stores, particularly that in skeletal muscles, are essentially reduced to nitrite and NO for vasodilation during high-intensity exercise (43). Our athlete subjects were routinely trained at high intensity. Indeed, their nitrate store was more depleted than that in the non-athlete controls (Table 2). Thus, from our results, even young athletes will need nitrate supplementation more than non-athletes of similar age, not just for sports performance but also for their vascular health (43).
Limitation
This work nonetheless is limited by the small sample size for a FMD study (44). The main reason is the low prevalence of GP.Mur blood type in northern Taiwan (<2%) where this study was conducted. To minimize variations among subjects, we recruited only male elite athletes from top sports universities in the region. But this type of study may be even harder to conduct in the future, as we have noticed gradual dilution of the GYP.Mur gene in Taiwan in the past half century.
Data Availability Statement
The raw data supporting the conclusions of this article will be made available by the authors, without undue reservation.
Ethics Statement
The studies involving human participants were reviewed and approved by MMH-Institutional Review Board (IRB registration: 19MMHIS081e). The patients/participants provided their written informed consent to participate in this study.
Author Contributions
KH, Y-YL, and W-CT conceived the project. W-CT and K-WT recruited the subjects. Y-YL, KH, and H-JL performed ultrasound experiments. C-YL supported ultrasound experiments. L-YC, H-LL, and H-JL performed blood tests and analyzed the data. K-TH helped conceptualize the data. H-IY edited the paper. KH analyzed the data and wrote the paper. All authors contributed to the article and approved the submitted version.
Funding
This work was supported by grants from Taiwan Ministry of Science & Technology (MOST 108-2628-B-195-001; MOST 109-2628-B-195-001; MOST 110-2628-B-195-001), and MacKay Memorial Hospital to KH (MMH 108-149; MMH 109-21; MMH 110-25; MMH 111-26, TTMMH 107-06; TTMMH 109-04).
Conflict of Interest
The authors declare that the research was conducted in the absence of any commercial or financial relationships that could be construed as a potential conflict of interest.
Publisher's Note
All claims expressed in this article are solely those of the authors and do not necessarily represent those of their affiliated organizations, or those of the publisher, the editors and the reviewers. Any product that may be evaluated in this article, or claim that may be made by its manufacturer, is not guaranteed or endorsed by the publisher.
Acknowledgments
We thank Ms. Yen-Ting Lin (Hitachi Medical Taiwan Ltd., Taiwan) for FMD support, and athlete and non-athlete subjects from the University of Taipei and MacKay Medical College for participating in this study.
References
1. Premont RT, Reynolds JD, Zhang R, Stamler JS. Role of nitric oxide carried by hemoglobin in cardiovascular physiology: developments on a three-gas respiratory cycle. Circ Res. (2020) 126:129–58. doi: 10.1161/CIRCRESAHA.119.315626
2. Lundberg JO, Weitzberg E, Gladwin MT. The nitrate-nitrite-nitric oxide pathway in physiology and therapeutics. Nat Rev Drug Discov. (2008) 7:156–67. doi: 10.1038/nrd2466
3. Bailey DM, Rasmussen P, Overgaard M, Evans KA, Bohm AM, Seifert T, et al. Nitrite and S-Nitrosohemoglobin exchange across the human cerebral and femoral circulation: relationship to basal and exercise blood flow responses to hypoxia. Circulation. (2017) 135:166–76. doi: 10.1161/CIRCULATIONAHA.116.024226
4. Tejero J, Shiva S, Gladwin MT. Sources of vascular nitric oxide and reactive oxygen species and their regulation. Physiol Rev. (2019) 99:311–79. doi: 10.1152/physrev.00036.2017
5. Cosby K, Partovi KS, Crawford JH, Patel RP, Reiter CD, Martyr S, et al. Nitrite reduction to nitric oxide by deoxyhemoglobin vasodilates the human circulation. Nat Med. (2003) 9:1498–505. doi: 10.1038/nm954
6. Huang KT, Han TH, Hyduke DR, Vaughn MW, Van Herle H, Hein TW, et al. Modulation of nitric oxide bioavailability by erythrocytes. Proc Natl Acad Sci USA. (2001) 98:11771–6. doi: 10.1073/pnas.201276698
7. Vitturi DA, Teng X, Toledo JC, Matalon S, Lancaster JR Jr, Patel RP. Regulation of nitrite transport in red blood cells by hemoglobin oxygen fractional saturation. Am J Physiol Heart Circ Physiol. (2009) 296:H1398–407. doi: 10.1152/ajpheart.01303.2008
8. Pawloski JR, Hess DT, Stamler JS. Export by red blood cells of nitric oxide bioactivity. Nature. (2001) 409:622–6. doi: 10.1038/35054560
9. Gladwin MT, Raat NJ, Shiva S, Dezfulian C, Hogg N, Kim-Shapiro DB, et al. Nitrite as a vascular endocrine nitric oxide reservoir that contributes to hypoxic signaling, cytoprotection, and vasodilation. Am J Physiol Heart Circ Physiol. (2006) 291:H2026–35. doi: 10.1152/ajpheart.00407.2006
10. Walder JA, Chatterjee R, Steck TL, Low PS, Musso GF, Kaiser ET, et al. The interaction of hemoglobin with the cytoplasmic domain of band 3 of the human erythrocyte membrane. J Biol Chem. (1984) 259:10238–46: doi: 10.1016/S0021-9258(18)90956-7
11. Hsu K, Lin YC, Chao HP, Lee TY, Lin M, Chan YS. Assessing the frequencies of GP.Mur (Mi.III) in several Southeast Asian populations by PCR typing. Transfus Apher Sci. (2013) 49:370–1. doi: 10.1016/j.transci.2013.05.011
12. Broadberry RE, Lin M. The distribution of the MiIII (Gp.Mur) phenotype among the population of Taiwan. Transfus Med. (1996) 6:145–8. doi: 10.1046/j.1365-3148.1996.d01-64.x
13. Huynh NT, Ford DS, Duyen TT, Huong MT. Jk and Mi.III phenotype frequencies in North Vietnam. Immunohematology. (2003) 19:57–8. doi: 10.21307/immunohematology-2019-476
15. Huang CH, Blumenfeld OO. Molecular genetics of human erythrocyte MiIII and MiVI glycophorins. use of a pseudoexon in construction of two delta-alpha-delta hybrid genes resulting in antigenic diversification. J Biol Chem. (1991) 266:7248–55. doi: 10.1016/S0021-9258(20)89637-9
16. Groves JD, Tanner MJ. The effects of glycophorin A on the expression of the human red cell anion transporter (band 3) in Xenopus oocytes. J Membr Biol. (1994) 140:81–8. doi: 10.1007/BF00234488
17. Groves JD, Tanner MJ. Glycophorin A facilitates the expression of human band 3-mediated anion transport in Xenopus oocytes. J Biol Chem. (1992) 267:22163–70. doi: 10.1016/S0021-9258(18)41649-3
18. Hsu K, Chi N, Gucek M, Van Eyk JE, Cole RN, Lin M, et al. Miltenberger blood group antigen type III (Mi.III) enhances the expression of band 3. Blood. (2009) 114:1919–28. doi: 10.1182/blood-2008-12-195180
19. Reithmeier RA. A membrane metabolon linking carbonic anhydrase with chloride/bicarbonate anion exchangers. Blood Cells Mol Dis. (2001) 27:85–9. doi: 10.1006/bcmd.2000.0353
20. Reithmeier RA, Casey JR, Kalli AC, Sansom MS, Alguel Y, Iwata S. Band 3, the human red cell chloride/bicarbonate anion exchanger (AE1, SLC4A1), in a structural context. Biochim Biophys Acta. (2016) 1858:1507–32. doi: 10.1016/j.bbamem.2016.03.030
21. Hsu K. Exploring the potential roles of band 3 and Aquaporin-1 in Blood CO2 transport-inspired by comparative studies of glycophorin B-A-B hybrid protein GP.Mur. Front Physiol. (2018) 9:733. doi: 10.3389/fphys.2018.00733
22. Hsu K, Kuo MS, Yao CC, Lee TY, Chen YC, Cheng HC, et al. Expedited CO2 respiration in people with Miltenberger erythrocyte phenotype GP.Mur. Sci Rep. (2015) 5:10327. doi: 10.1038/srep10327
23. Hsu K, Lin HJ, Yao CC, Cheng HC, Kuo MS. Borderline high blood pressure in people with Miltenberger GP.Mur phenotype is associated with elevated band 3 expression on the erythrocyte membrane. FASEB J. (2016) 30 (Suppl):lb76. doi: 10.1096/fasebj.30.1_supplement.lb76
24. Burton NM, Bruce LJ. Modelling the structure of the red cell membrane. Biochem Cell Biol. (2011) 89:200–15. doi: 10.1139/O10-154
25. Hsu K, Lee TY, Chao HP, Chan YS, Lin YC, Lin M. Expression of the Rh/RhAG complex is reduced in Mi.III erythrocytes. Vox Sang. (2012) 102:221–7. doi: 10.1111/j.1423-0410.2011.01535.x
26. Hsu K, Lee TY, Periasamy A, Kao FJ, Li LT, Lin CY, et al. Adaptable interaction between aquaporin-1 and band 3 reveals a potential role of water channel in blood CO2 transport. FASEB J. (2017) 31:4256–64. doi: 10.1096/fj.201601282R
27. Hsu K, Kuo MS, Yao CC, Cheng HC, Lin HJ, Chan YS, et al. The MNS glycophorin variant GP.Mur affects differential erythroid expression of Rh/RhAG transcripts. Vox Sang. (2017) 112:671–7. doi: 10.1111/vox.12555
28. Schuck P, Schubert D. Band 3-hemoglobin associations. the band. 3 tetramer is the oxyhemoglobin binding site. FEBS Lett. (1991) 293:81–4. doi: 10.1016/0014-5793(91)81156-3
29. Hsu K, Lin YC, Chang YC, Chan YS, Chao HP, Lee TY, et al. A direct blood polymerase chain reaction approach for the determination of GP.Mur (Mi.III) and other Hil+ Miltenberger glycophorin variants. Transfusion. (2013) 53:962–71. doi: 10.1111/j.1537-2995.2012.03861.x
30. Leftheriotis G. Flow mediated vasodilatation a way to evaluate vascular healthiness with eTRACKING technology. In: ALOKA Science and Humanity-Prosound (2008).
31. Allen JD, Cobb FR, Gow AJ. Regional and whole-body markers of nitric oxide production following hyperemic stimuli. Free Radic Biol Med. (2005) 38:1164–9. doi: 10.1016/j.freeradbiomed.2004.12.018
32. Bonini MG, Stadler K, Silva SO, Corbett J, Dore M, Petranka J, et al. Constitutive nitric oxide synthase activation is a significant route for nitroglycerin-mediated vasodilation. Proc Natl Acad Sci USA. (2008) 105:8569–74. doi: 10.1073/pnas.0708615105
33. Tremblay JC, Hoiland RL, Howe CA, Coombs GB, Vizcardo-Galindo GA, Figueroa-Mujica RJ, et al. Global REACH 2018, high blood viscosity and hemoglobin concentration contribute to reduced flow-mediated dilation in high-altitude excessive erythrocytosis. Hypertension. (2019) 73:1327–35. doi: 10.1161/HYPERTENSIONAHA.119.12780
34. Kleinbongard P, Dejam A, Lauer T, Rassaf T, Schindler A, Picker O, et al. Plasma nitrite reflects constitutive nitric oxide synthase activity in mammals. Free Radic Biol Med. (2003) 35:790–6. doi: 10.1016/S0891-5849(03)00406-4
35. Lundberg JO, Weitzberg E. NO generation from nitrite and its role in vascular control. Arterioscler Thromb Vasc Biol. (2005) 25:915–22. doi: 10.1161/01.ATV.0000161048.72004.c2
36. Hsu K, Lin YC, Lee TY, Lin M. Miltenberger blood group antigen subtype III (Mi.III) supports Wr(b) expression. Vox Sang. (2011) 100:389–94. doi: 10.1111/j.1423-0410.2010.01436.x
37. Pyke K, Green DJ, Weisbrod C, Best M, Dembo L, O'Driscoll G, et al. Nitric oxide is not obligatory for radial artery flow-mediated dilation following release of 5 or 10 min distal occlusion. Am J Physiol Heart Circ Physiol. (2010) 298:H119–26. doi: 10.1152/ajpheart.00571.2009
38. Green DJ, Dawson EA, Groenewoud HM, Jones H, Thijssen DH. Is flow-mediated dilation nitric oxide mediated?: a meta-analysis. Hypertension. (2014) 63:376–82. doi: 10.1161/HYPERTENSIONAHA.113.02044
39. Krams R, Bäck M, European Society of Cardiology. The ESC Textbook of Vascular Biology. Oxford: Oxford University Press (2017) 295. doi: 10.1093/med/9780198755777.001.0001
40. Herrera M, Hong NJ, Garvin JL. Aquaporin-1 transports NO across cell membranes. Hypertension. (2006) 48:157–64. doi: 10.1161/01.HYP.0000223652.29338.77
41. Herrera M, Garvin JL. Aquaporins as gas channels. Pflugers Arch. (2011) 462:623–30. doi: 10.1007/s00424-011-1002-x
42. Herrera M, Garvin JL. Novel role of AQP-1 in NO-dependent vasorelaxation. Am J Physiol Renal Physiol. (2007) 292:F1443–51. doi: 10.1152/ajprenal.00353.2006
43. Wylie LJ, Park JW, Vanhatalo A, Kadach S, Black MI, Stoyanov Z, et al. Human skeletal muscle nitrate store: influence of dietary nitrate supplementation and exercise. J Physiol. (2019) 597:5565–76. doi: 10.1113/JP278076
44. Corretti MC, Anderson TJ, Benjamin EJ, Celermajer D, Charbonneau F, Creager MA, et al. International Brachial Artery Reactivity Task, guidelines for the ultrasound assessment of endothelial-dependent flow-mediated vasodilation of the brachial artery: a report of the International Brachial Artery Reactivity Task Force. J Am Coll Cardiol. (2002) 39:257–65. doi: 10.1016/S0735-1097(01)01746-6
Keywords: flow-mediated vasodilation (FMD), nitric oxide (NO), Band 3, GP.Mur, red blood cell (RBC), hemoglobin (Hb), nitrite, athletes
Citation: Hsu K, Liu Y-Y, Tseng W-C, Huang K-T, Liu C-Y, Chen L-Y, Lee H-L, Lin H-J, Tseng K-W and Yeh H-I (2021) Comodulation of NO-Dependent Vasodilation by Erythroid Band 3 and Hemoglobin: A GP.Mur Athlete Study. Front. Cardiovasc. Med. 8:740100. doi: 10.3389/fcvm.2021.740100
Received: 12 July 2021; Accepted: 05 November 2021;
Published: 29 November 2021.
Edited by:
Lee Stoner, University of North Carolina at Chapel Hill, United StatesReviewed by:
Palash Chandra Banik, Bangladesh University of Health Sciences, BangladeshTatiana Vavilova, Almazov National Medical Research Centre, Russia
Copyright © 2021 Hsu, Liu, Tseng, Huang, Liu, Chen, Lee, Lin, Tseng and Yeh. This is an open-access article distributed under the terms of the Creative Commons Attribution License (CC BY). The use, distribution or reproduction in other forums is permitted, provided the original author(s) and the copyright owner(s) are credited and that the original publication in this journal is cited, in accordance with accepted academic practice. No use, distribution or reproduction is permitted which does not comply with these terms.
*Correspondence: Kate Hsu, khsu1@mmh.org.tw; orcid.org/0000-0003-1009-0997
†These authors share first authorship