Heat Tolerance, Energetics, and Thermal Treatments of Honeybees Parasitized With Varroa
- 1Facultad de Agronomía e Ingeniería Forestal, Pontificia Universidad Católica de Chile, Santiago, Chile
- 2Departamento de Ecología, Center of Applied Ecology and Sustainability, Facultad de Ciencias Biológicas, Pontificia Universidad Católica de Chile, Santiago, Chile
Ongoing global change affects both wildlife and economically relevant species, which are now subjected to combined challenges from climate change and higher exposure to pathogens. Honeybee colonies worldwide are under threat by higher temperatures and the ectoparasitic mite Varroa destructor, hence we studied the impact of these combined challenges in the thermal biology and energetics of Apis mellifera. We estimated the heat tolerance and energy expenditure (CO2 production and VCO2) of honeybees acclimated to different temperatures (32 and 38°C) and subjected to different levels of parasitism (0, 1, and 2 mites). Heat tolerance was quantified employing thermal death time (TDT) curves describing how survival times vary as a function of temperature, which differed significantly between treatments. Warm-acclimated uninfected bees exhibited a higher thermal tolerance than their cold-acclimated counterparts, but parasitism by Varroa resulted in a substantial drop in tolerance rendering TDT curves of parasitized bees virtually indistinguishable. Accordingly, VCO2 increased dramatically in parasitized bees (46.5 and 67.1% with 1 and 2 Varroa, respectively), suggesting that Varroa impinges on substantial costs on energy expenditure which, in combination with lower fat reserves due to parasitism, should have synergistic effects on bees’ survival and performance. Results provide conclusive evidence of the detrimental impact of Varroa on heat tolerance that undermines potentially adaptive responses associated with thermal acclimation. Results also show that heat treatments are a realistic venue to control Varroa, and we discuss how TDT curves may be employed to optimize management strategies in this context.
Introduction
Studies of thermal biology in insect-parasite interactions have shown that resistance, host recovery, pathogen virulence, and replication can be significantly altered by temperature (Schmidt-Hempfel, 2008, 2009), suggesting that the thermal environment could have profound implications for host/parasite dynamics, and co-evolution (see Thomas and Blanford, 2003). The reported population declines of honeybees (Apis mellifera) in different regions of the globe constitute a paramount example of this problem (Neumann and Carreck, 2010; Ratnieks and Carreck, 2010; Kang et al., 2016; Maggi et al., 2016; Nazzi and Le Conte, 2016; Requier et al., 2018; Ramsey et al., 2019), with potentially important repercussions on crop and seed production in agricultural ecosystems (Mandrioli, 2012; Nazzi and Le Conte, 2016). For example, changes in weather conditions are provoking new pest invasion to crops, deforestation, shortened flowering seasons or changes in phenology of plants, and insects, among others (Klein et al., 2017). On the other hand, when the brood is exposed to extreme cold or warm temperatures, it could affect the behavior in workers when they are foragers (Tautz et al., 2003; Jones et al., 2004; Becher et al., 2009).
Several authors argue that these losses are caused by the ectoparasitic mite Varroa destructor (Acari:Mesostigmata), the most common pest in beekeeping that is responsible for the disease varroosis, and present in virtually every country where the Western bee is found with few exceptions (Nazzi and Le Conte, 2016; Ramsey et al., 2019). This mite feeds directly from the host, consuming their fatty acids or lipids and the hemolymph from immature and adult bees (Richards et al., 2011; Ramsey et al., 2019), and acts as a vector of viruses, bacteria, and fungi (Annoscia et al., 2012; Riveros et al., 2019). A wide range of morphological and physiological changes have been reported in adult honeybees parasitized during their metamorphosis phase, such as a lower body weight, body, and appendices deformities, decreased longevity, depression of the immune system, changes in the cuticular hydrocarbons profiles, and reduction in hemolymphatic proteins (Lee et al., 2010; Schäfer et al., 2010; Annoscia et al., 2012; Erban et al., 2019). Without treatment, most of the colonies in temperate regions collapse in one to 3 years (Fries et al., 2006; Rosenkranz et al., 2010; Ramsey and van Engelsdorp, 2016). Overall, some estimations suggest that Varroa has provoked more damage to honeybee colonies than all other known honeybee diseases and parasites combined (Emsen et al., 2015; Maggi et al., 2016; Evans and Cook, 2018).
On top of that, honeybee colonies around the globe are being exposed to increasingly higher temperatures due to global warming, and which can have detrimental effects for multiple reasons. For instance, higher thermal averages and extremes may affect the honeybees’ thermal performance, constrain their activity periods, or increase water loss rates, all of which might affect survival and have a direct effect on colony stability (Annoscia et al., 2012; Mandrioli, 2012; Klein et al., 2017). In addition, energetic trade-offs associated with sublethal temperatures may have an impact on immune function and render these colonies more susceptible to infection (Schmidt-Hempfel, 2009) or, instead, increase the energy and water requirements of the hive (Aldea and Bozinovic, 2020). And finally, from a pathogen’s or parasite’s perspective, higher temperatures may have a positive effect on thermal performance and effectively promote or facilitate biological invasions by pests (Cornelissen et al., 2019). Needless to say, determining how changes in the thermal environment may affect the interaction between Apis and Varroa, as well as its impact on metabolic and thermal performance, and is of paramount importance to determine how honeybee colonies might respond to different climate forecasts in the future (Kovac et al., 2007, 2014).
Here we address this issue, focusing on the impact of different parasitic loads of Varroa on the survival and energy expenditure of adult honeybee workers. We study adult bees because the evidence of detrimental effects of mites in this life stage remains limited and ambiguous (Nazzi and Le Conte, 2016), even though it has been speculated that the consumption of fat reserves by mites should significantly reduce energy storage and affect the immune response (Robar et al., 2011; Ramsey et al., 2019). Specifically, we quantified the putative impact of thermal history in combination with a variable parasitic load on heat tolerance and energy expenditure of honeybees everything else being equal. We hypothesized that bees maintained at higher acclimation temperatures would be more sensitive to Varroa infestation and exhibit more pronounced detrimental effects of this ectoparasite than their counterparts maintained at less stressful temperatures.
Materials and Methods
Honeybees (hybrid from A. m. ligustica and A. m. carnica) were kept in an apiary located in Mediterranean agroecosystems of Central Chile (34°03′S and 70°41′W) with six colonies. In each colony, the queen mated naturally at the beginning of the season (early in September). All the colonies were standardized with ten frames with bees, three frames with open brood, and four with sealed brood and three with food (honey and pollen). This apiary had a strict sanitary control for all diseases with organic treatment (Oxalic acid), especially against Varroa, and to ensure that we have healthy bees with an infestation level under 2% of mites (two phoretic mites per one hundred of worker bees). A second apiary with three colonies, maintained in our laboratory (33°22′S and 70°36′W), was employed as a source of Varroa. In this case no sanitary control was applied, and workers or drone brood production was stimulated to obtain a high number of mites from each frame. Each month during the late Austral spring and summer of 2017 and 2018 (between October and March), we allowed the queen to lay eggs in one empty comb per colony for 10 days, and then she was removed from the hive. Subsequently, we moved worker and drone brood frames from the healthy and parasitized colonies, respectively, into two climatic chambers with ambient temperatures (Ta) of 32 or 38 ± 1.2°C, humidity of 55 ± 5%, and photoperiod of L:D = 0:24 (Crailsheim et al., 2012; Hartfelder et al., 2013). We used 32°C as acclimation temperature in the control group according to rearing bee methodology described by Crailsheim et al. (2012) and Medrzycki et al. (2010), and we chose 38°C as a warmer temperature of acclimation according to extreme temperature registered inside colonies in the field during late summer (unpublished data). The worker brood was maintained in the chambers as sealed brood for 5 or 6 days. In the case of 32°C degrees group, acclimation temperature was reached immediately but, in the case of 38°C degrees group, acclimation temperature started at 32°C degrees, and it was increasing 0.5°C per 180 min until the chamber reached 38°C. Emerged bees were kept in small plastic units grouped randomly (∼100 individuals) and fed with 50% of sugar syrup solution and a honeybee commercial aminoacids/vitamins product (Promotor L®) for 6 to 10 days before the experiments. Summarizing, bees were acclimated for a period between 11 and 16 days. In parallel, we maintained in a separate climatic chamber at 32 ± 1.2°C the infested brood with Varroa (Dietemann et al., 2013).
To carry out the heat tolerance and metabolic assays with different loads of parasites (0, 1, or 2 Varroa per honeybee), we collected mites from infected brood and maintained them in Petri dishes for at least 3 h at 32°C before transplanting them onto individual healthy honeybees (below). This protocol ensured that mites would feed on their newly transferred host prior to measurements (Dietemann et al., 2013), resulting in an experimental design with control groups at each acclimation temperature (32 and 38°C) that were not infected, and experimental groups with contrasting parasitic loads. Before each assay, all the bees were weighed, and we used bees between 85 and 135 mg of mass (see Supplementary Material).
Heat Tolerance
We employed thermal death time (TDT) curves to estimate heat tolerance, as originally proposed by Rezende et al. (2014). This approach discriminates between the intensity and the duration of a thermal stress, which are confounded in assays with rising temperatures, indicating how organism might respond to an acute thermal challenge versus chronic exposition to fewer extreme temperatures (Rezende et al., 2014). Succinctly, TDT curves can be described with the following relationship:
where, T corresponds to the lethal temperature (°C), CTmax to the temperature resulting in death after a 1-min exposure (°C), z to the temperature required to change the survival time in one order of magnitude (°C) and t the time to death (min). Note that CTmax and z resemble the intercept and slope of a linear regression, and for sake of simplicity, a CTmax = 40°C and z = 3°C would imply that an organism would tolerate 37°C for 10 min, 34°C for 100 min and so on (Rezende et al., 2014). This is standard methodology and has been currently shown to be applicable to predict thermal mortality in fluctuating environments (Rezende et al., 2020).
We estimated TDT curves as implemented by Castañeda et al. (2015), placing mites with the honeybees prior to assays. We collected adult bees younger than 9 days, weighed each individual in an analytical balance (0.1 mg; JK-180, Chiyo, Kyoto), and placed one or two mites directly onto the bee with a brush and left them in an Eppendorf tube inside the climatic chamber for 1 h to ensure effective parasitism. For the control groups, we replicated the manipulation of the honeybees with the brush but did not place any mite onto them. Then, we measured heat tolerance for 60 individuals simultaneously in a water bath (46 cm × 35 cm × 35 cm) containing a rack with 4 rows × 15 columns of vials, with 15 individuals per treatment randomized within each bath. We used constant temperatures of 45, 47, 49, and 51°C, which resulted in assays lasting no more than 3 h, and two replicate baths per temperature. Water temperature was controlled by a programmable heating unit that also ensured proper water circulation (JULABO ED, JULABO Labortechnik, Seelbach, Germany). The behavior of each bee was recorded using a digital HD video camera (SONY HDR-CX110E, Tokyo, Japan), and the time to death t was estimated as the period required for each individual to lose motor coordination or activity to cease. With this design, heat tolerance trials involved a total n = 720 honeybees (=15 individuals × 4 measurement temperatures × 2 baths × 2 acclimation temperatures × 3 parasitic loads) and the same number of Varroa.
Metabolic Rate
Before metabolic trials, we weighed each individual and randomly assigned them to one of the three Varroa treatments as described above for the heat tolerance assays. Here, measurements involved ten bees per treatment, resulting in a total n = 60 (10 individuals × 2 acclimations × 3 parasitic loads), and individuals were considered to be at rest only if “no or only small visible signs of activity like small movements of antennae or single legs” were observed (Kovac et al., 2007). We measured rates of CO2 production (VCO2) in a glass metabolic chamber using an open-flow system (Sable Systems), following Lighton (2008), and Lighton and Halsey (2011). Each honeybee in the metabolic chamber was placed inside a temperature-controlled incubator, and measurements were performed for 3 h at the same temperature in which they were acclimated. Airflow was set to 150 ml/min, the CO2 was scrubbed from the air with a Drierite column before entering the chamber, and VCO2 was continuously recorded (Lighton, 2008). Data were transformed from percentage to volume per min and total CO2 production was calculated with EXPEDATA (Sable Systems).
Statistical Analyses
Statistical analyses were performed using the STATISTICA® (2001) version 6.0 statistical package for Windows® operative system and R1. Analyses involved generalized linear models (GLM) to compare body size across acclimation temperatures, and then to compare heat tolerance and metabolic rates between treatments. Survival times at each temperature were compared employing a 2-factor ANOVA including acclimation and levels of parasitism as diagnostics for subsequent TDT analyses. Calculation of the TDT curve parameters was performed with separate linear regressions for each treatment, between measurement temperature T vs. log10 t (Equation 1), followed by the back-transformation CTmax = – intercept/slope, and z = 1/slope to ensure that analyses are performed with the appropriate minimum sums of squares (Rezende et al., 2014). Standard errors for CTmax and z were estimated numerically from the error propagation of regression coefficient estimates, taking into consideration that slope and intercept are negatively correlated. We also quantified survival probability curves during heat tolerance challenges for all treatments as described in Castañeda et al. (2015). In these survival curves, the elapsed time t required for 50% mortality tends to exhibit the semi-logarithmic relationship with temperature T described by TDT curves (Equation 1). Differences in heat tolerance across treatments were assessed with two complementary approaches. First, with a generalized linear model including log10 t as the dependent variable varying as a function of T, acclimation temperature, parasitic load (0, 1, and 2 Varroa) and log10 body mass:
Parasitic load Var was included as a factor (2 df) because preliminary analyses showed that the effects of the number of Varroa were non-additive. Differences in elevation between acclimation temperatures and levels of parasitism were estimated with main effects, whereas differences in slope were tested with the pairwise interaction between T and these terms (Equation 2). We did not control for replicate bath because the treatments were balanced and randomized within each bath (see section “Heat Tolerance”). Second, we compared the estimated 95% confidence intervals of parameters CTmax and z, and contrasted these results against the outcome of the GLM. For metabolic rates, we employed a similar GLM including only Tacc, Var and log10 mass.
Results
In both sets of honeybees employed for the heat tolerance and metabolic assays, a two way ANOVA showed that acclimation temperature had a significant impact on body mass (F1,716 = 618.1, p < < 0.0001) with a warm acclimation temperature resulting in lighter individuals (121.5 ± 14.7 mg at 32°C, and 93.5 ± 15.2 mg at 38°C for bees in the heat tolerance assays) (mean ± SD), whereas no significant differences were detected across the Varroa treatments (F1,716 = 1.23, p = 0.291). Therefore, while analyses subsequent analyses are performed controlling for body mass, it is important to recall that the effects of acclimation temperatures in heat tolerance and metabolic rates can be partitioned into direct acclimation responses in heat tolerance and metabolic rates, and the indirect effects mediated by changes in body mass.
With regards to thermal tolerance, survival probability curves (Figure 1) and comparisons between TDT curves (Figure 2) show that heat tolerance is affected by both acclimation temperatures and levels of parasitism (Table 1). The significant interaction detected between these factors (Table 1), concomitantly with the similar tolerance between Varroa treatments at 32°C (Figure 2), and suggest that the effect of parasitism is prevalent in animals acclimated to 38°C. In this group, survival probability curves indicate that 1 or 2 Varroa have a conspicuous effect in mortality rates at less extreme temperatures, particularly at 45 and 47°C, whereas at higher temperatures the impact of heat stress prevail, and survival curves obtained across honeybees subjected to different levels of parasitism become virtually indistinguishable (Figure 1). These results are mirrored by the TDT curve analysis (Figure 2), where we detected significant differences between elevation and slopes of curves as a function of acclimation temperature (F1,711 = 8.67, p = 0.0033 and F1,711 = 7.38, p = 0.0067 for the intercept and slope, respectively) and levels of parasitism (F2,711 = 4.30, p = 0.014 and F2,711 = 3.61, p = 0.028). Estimates of CTmax indicate that TDT curves at 32°C are shifted downward with respect to curves at 38°C (Figure 2), whereas estimates of z are generally lower in honeybees acclimated to 38°C and show that the increase in death times at less extreme temperatures is disproportionally higher in this group (Figure 2). These results indicate that warm-acclimated honeybees exhibit a higher tolerance to nearly lethal and sublethal temperatures than their cold-acclimated counterparts. A closer inspection of this dataset indicates that Varroa parasitism reduces substantially survival times in bees acclimated at 38 but not at 32°C, and non-additive effects of multiple Varroa as suggested by preliminary analyses (Figure 2). Interestingly, log10 body mass was highly significant in the GLM (F1,711 = 34.3, p = 7.31 × 10–9), showing that larger individuals tended to collapse faster with a thermal challenge everything else being equal.
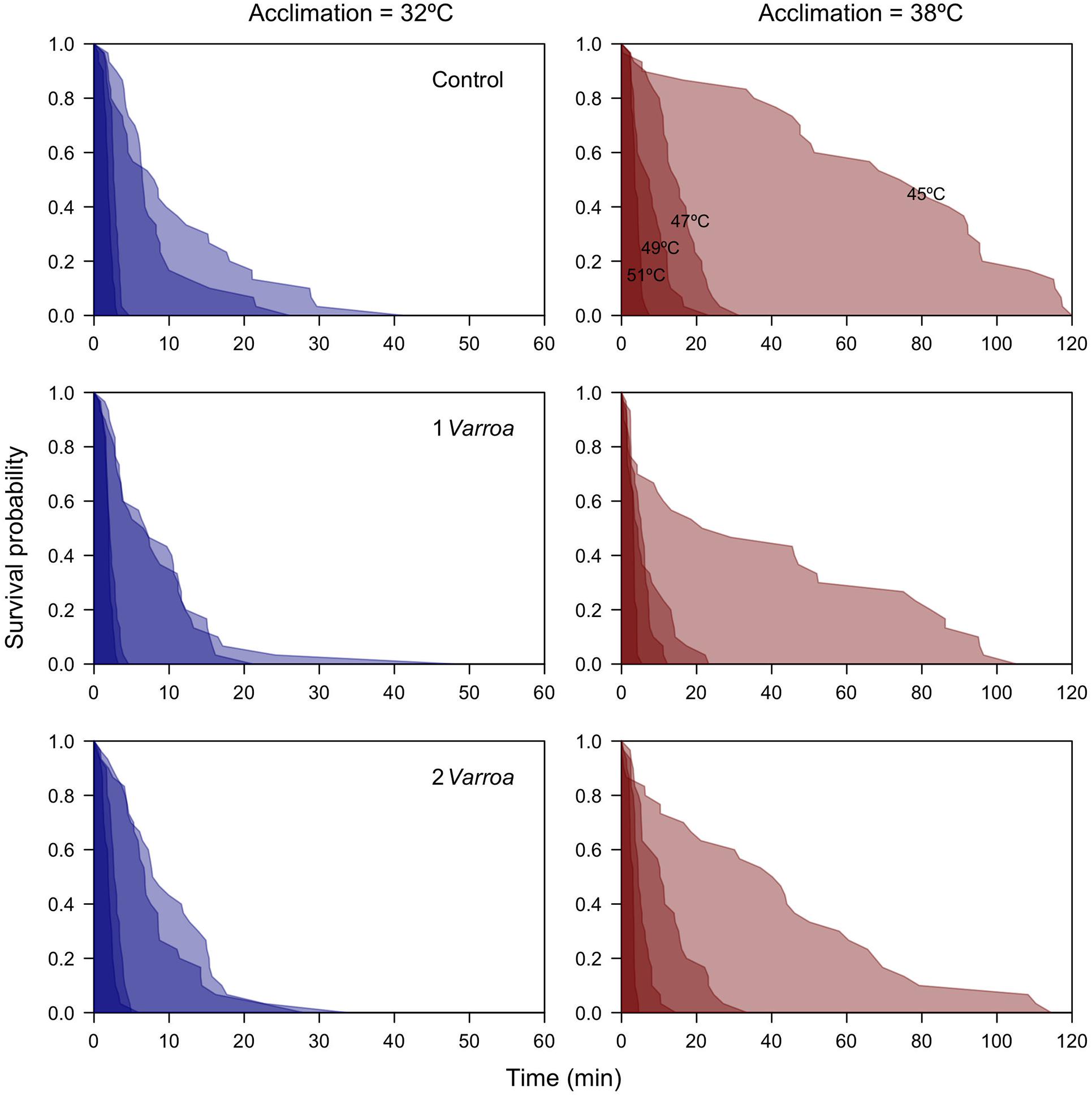
Figure 1. Survival curves obtained at different temperatures for honeybees acclimated to 32 and 38°C, and subjected to different levels of parasitism by Varroa (0, 1, and 2 mites). Measurements involved a total n = 720 individuals (n = 120 per panel).
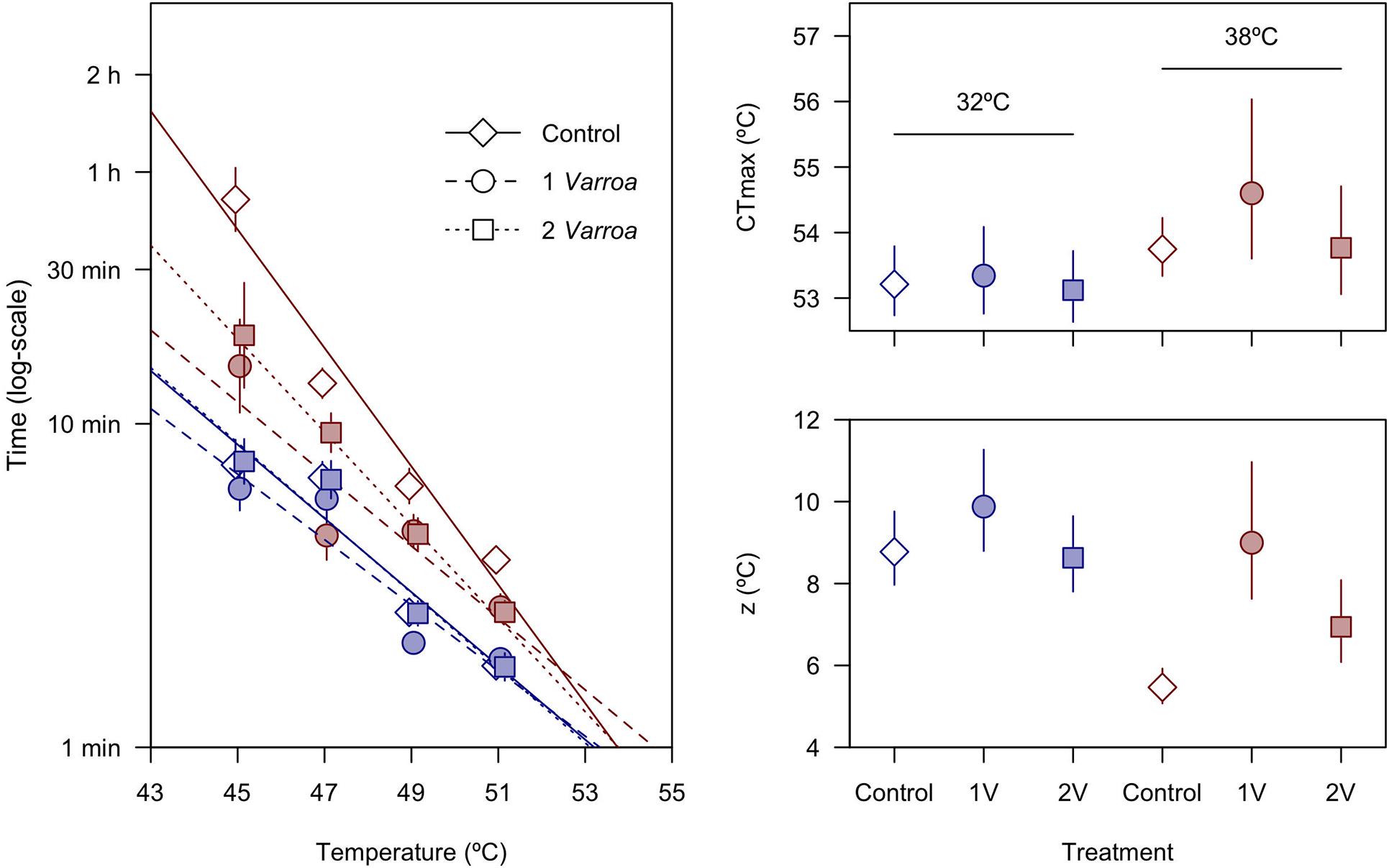
Figure 2. Heat tolerance in honeybees acclimated to 32 and 38°C and exposed to different levels of parasitism by Varroa, expressed as thermal death time (TDT) curves. Parameters CTmax and z represent, respectively, the thermal tolerance following an exposition of t = 1 min (i.e., the temperature that intercepts the abscissa) and the temperature difference required to increase t by one order of magnitude (see main text). Symbols in blue and red represent, respectively, acclimation temperatures of 32 and 38°C. Values are shown as mean ± SE.
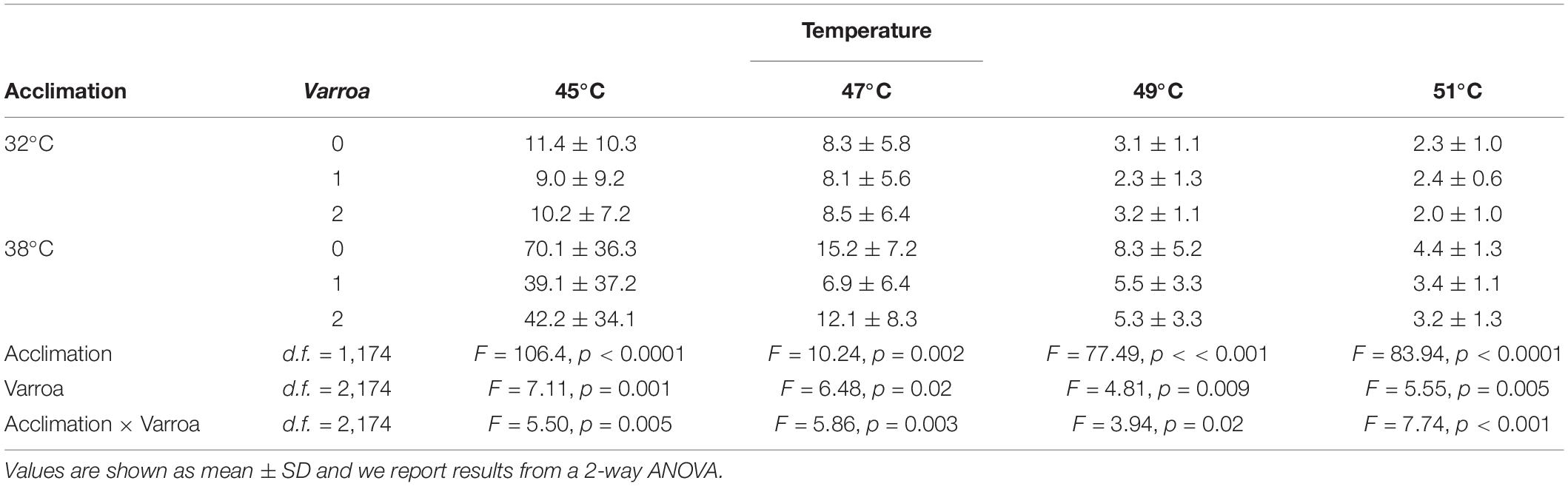
Table 1. Survival times (min) employed to estimate the thermal death time (TDT) curves for different treatments (n = 180 for each temperature).
With regards to energy expenditure, VCO2 was seemingly lower in the group acclimated to 38°C even after accounting for mass differences, suggesting that warm-acclimation results in a significant reduction in metabolism (Figure 3). Accordingly, in the GLM allometric effects were weak albeit significant (scaling exponent b = 0.607 ± 0.332, F1,55 = 3.35, 1-tailed p = 0.036) and thermal acclimation effects were negative (F1,55 = 10.74, p = 0.0018). These results mirrored by analyses with the control group alone. While the effects of parasitism in the full dataset were positive and significant (F1,55 = 9.29, p = 0.0003), suggesting that parasitized honeybees exhibit a higher VCO2 than control (Figure 3), this effect pools the responses of the honeybees as well as the metabolic contribution of Varroa. Partitioning these effects is not entirely straightforward, particularly because differences in VCO2 between treatments with 0, 1, and 2 Varroa show that effects are not additive: adjusted VCO2 = 2.52 ± 0.23 μL CO2/min, 3.69 ± 0.31 μL CO2/min and 4.21 ± 0.35 μL CO2/min, respectively ( ± SE). We performed a post hoc Tukey test between adjusted metabolic rates controlling for body mass to determine if there were significant between Varroa treatments (we did not perform pairwise comparisons across groups acclimated to different temperatures). With this approach, we detected significant differences between Control and bees with two Varroa at 32 and 38°C (1-tailed p = 0.0021 and 0.0473, respectively, see Supplementary Material). Assuming that the metabolism of Varroa is small to negligible given its small size when compared to adult bees, differences in adjusted means would indicate that parasitism with 1 and 2 Varroa increases VCO2 by, respectively, 46.5 and 67.1%. While metabolism increased with the number of Varroa and significant differences were detected between control and 2 Varroa, the other pairwise differences were not statistically significant (p ≥ 0.0995 in all cases).
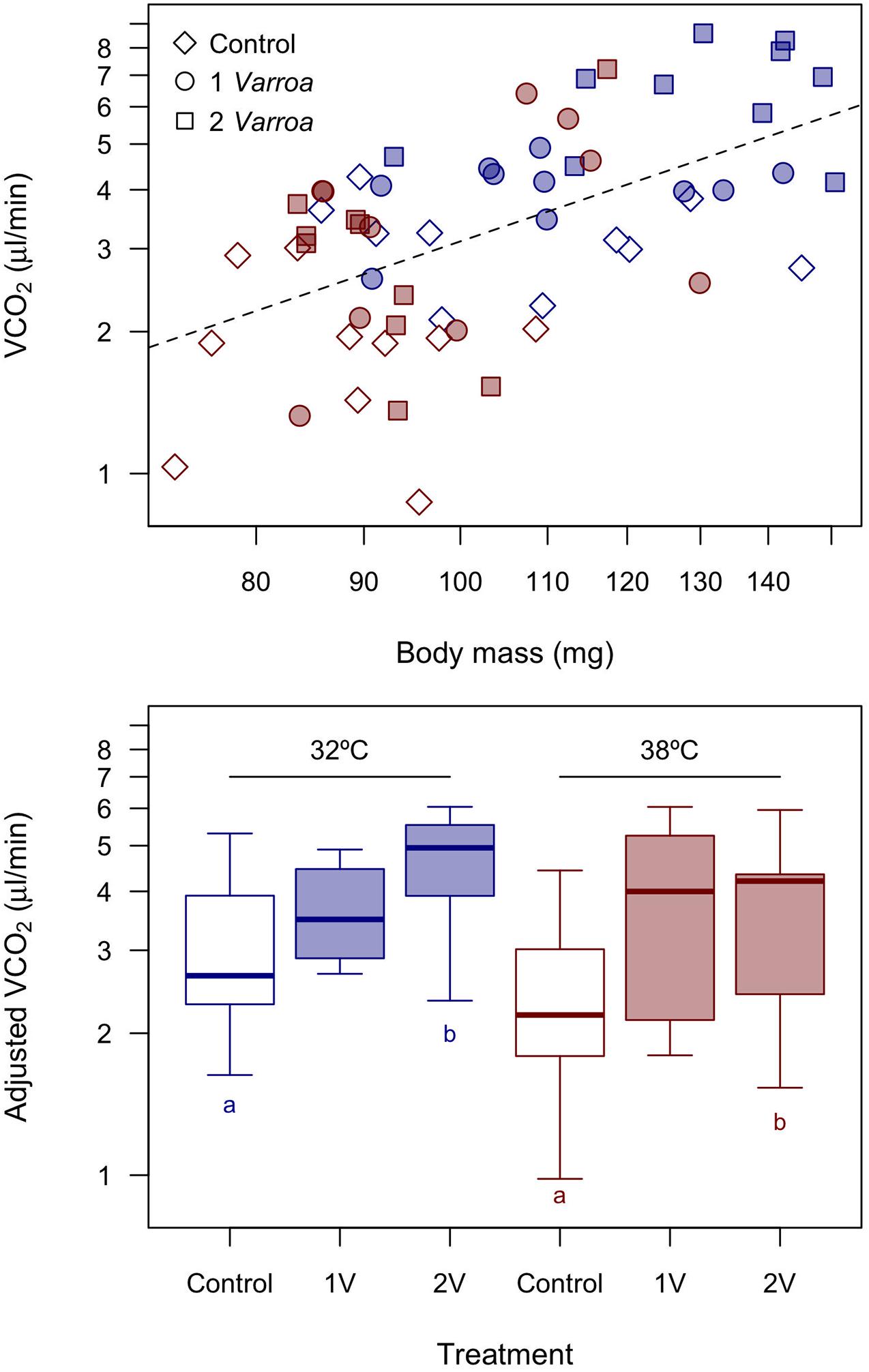
Figure 3. Metabolic rates in honeybees acclimated to 32 and 38°C and exposed to different levels of parasitism by Varroa. For the boxplot adjusted estimates were calculated for a body mass of 103.5 mg. Symbols in the scatterplot as in Figure 2. Pairwise differences between treatments employing Tukey contrasts (1-tailed p < 0.05) are shown with different letters (for simplicity we highlight only pairwise comparisons within acclimation groups, i.e., the effects of Varroa).
Discussion
Here we studied the effects of temperature acclimation and levels of parasitism in heat tolerance and energy expenditure of honeybees A. mellifera. Our results can be briefly summarized as follows. Bees acclimated to warmer temperatures exhibited a smaller size, higher thermal tolerance, and decreased metabolic rates than their cold-acclimated counterparts. Contrasting responses between control and parasitized individuals suggest that warm-acclimated honeybees are more susceptible to the impact of Varroa, presumably due to their smaller size and more restricted energy reserves. The increase in energy expenditure detected in parasitized individuals was substantial and, in combination with the removal of fat deposits in parasitized individuals, is expected to have synergistic detrimental effects. This might explain the high mortality rates observed during the beginning of the trials in parasitized honeybees, which are readily evident in the upper regions of the 45°C curves obtained following warm-acclimation (Figure 1). Many bees were collapsing at the onset of the trials, most likely due to distress associated with parasitism rather than the heat shock per se. A poor physiological condition, combined with the rise in temperature and the metabolic challenge that this entails, and likely explains this observation (Aldea and Bozinovic, 2020).
To our knowledge, this is the first estimation of TDT curves in healthy and parasitized honeybees, and results show that both acclimation history and Varroa have an impact on heat tolerance. Estimates of critical maximum temperatures obtained with ramping methods, where temperature increases at a constant rate, range between 44.6 and 51.8°C in different species of bees (Tan et al., 2005; Kovac et al., 2014; Hamblin et al., 2017), and which fall within the range we estimated for an acute thermal stress. However, differences in TDT curves suggest that acclimation and Varroa effects are particularly relevant during chronic exposure at less extreme temperatures (Figures 1, 2). With regards to thermal acclimation, estimates of CTmax and z for healthy individuals indicate that cold-acclimated bees can withstand 38°C for only 54 min (CTmax = 53.2°C, z = 8.77°C) whereas their warm-acclimated counterparts can tolerate this temperature for 750 min (CTmax = 53.7°C, z = 5.46°C) (calculations performed rearranging Equation 1). While the latter estimate is rather low considering that brood frames were maintained at 38°C, dehydration likely accounts for these lower survival times since bees had no access to food or water during TDT assays (Maynard-Smith, 1957; Rezende et al., 2011). Consequently, this result combined with the smaller size of honeybees raised at 38°C suggests that this acclimation temperature already imposes some degree of sublethal stress, which might partly explain why TDT curves for warm-acclimated honeybees were more highly affected by Varroa (Figure 2). For instance, while survival times estimated for a chronic exposure to 38°C is expected to decrease from 54 to 36 min in cold-acclimated bees exposed to one Varroa (CTmax = 53.3°C, z = 11.3°C), in warm-acclimated bees estimates drop from 760 min to roughly 32 min (CTmax = 54.6°C, z = 11.0°C). We ignore why detrimental effects were apparently stronger in individuals with one instead of two Varroa (Figure 2), but overall, these results indicate that Varroa can have a disproportional effect on bees subjected to higher temperatures, hence parasitism and thermal stress may have synergistic effects on survival and colony stability. This is evident in the warm-acclimated group, whereas in the cold-acclimated group the thermal stress may have been too strong (with 50% mortality attained in less than 10 min at all experimental temperatures), possibly overshadowing any potential impact of Varroa in heat tolerance.
With regards to energy expenditure, resting VCO2 in healthy individuals (Table 1) were like previous estimates of 2.14 μl/min at 32°C and 3.31 μl/min at 38°C (Kovac et al., 2007, 2014). As reported for some but not all host-parasite systems (see Robar et al., 2011), there was a marked increase in VCO2 in the treatments with Varroa indicating that the acute response to parasitism in honeybees is energetically expensive. Visual inspection suggests that the non-additive effects of Varroa in VCO2 were more pronounced in warm-acclimated bees, as observed for TDT curves, even though interactions between acclimation temperature and Varroa were not statistically significant possibly due to a restricted sample size in this experiment. Admittedly, the effects of body size, acclimation and measurement temperature are confounded, and it is considerably difficult to adequately tease them apart. Nonetheless, pairwise comparisons between treatments demonstrate that honeybees exhibit an enormous drop in metabolism in response to acclimation at 38°C, with VCO2 decreasing even when thermal effects are not considered (Table 2). While mass-specific VCO2 in non-parasite bees acclimated at 32 and 38°C and measured at these temperatures represent a 26.7% decrease in energy expenditure (Table 2), this amounts is a 57.7% drop after correcting for a Q10 = 2.5 and a 65.4% drop if differences in size are also considered. Despite the energy savings, it seems that this metabolic depression constitutes a stress response to elevated temperatures and, in the long run, would likely constraint activity, and locomotor performance.
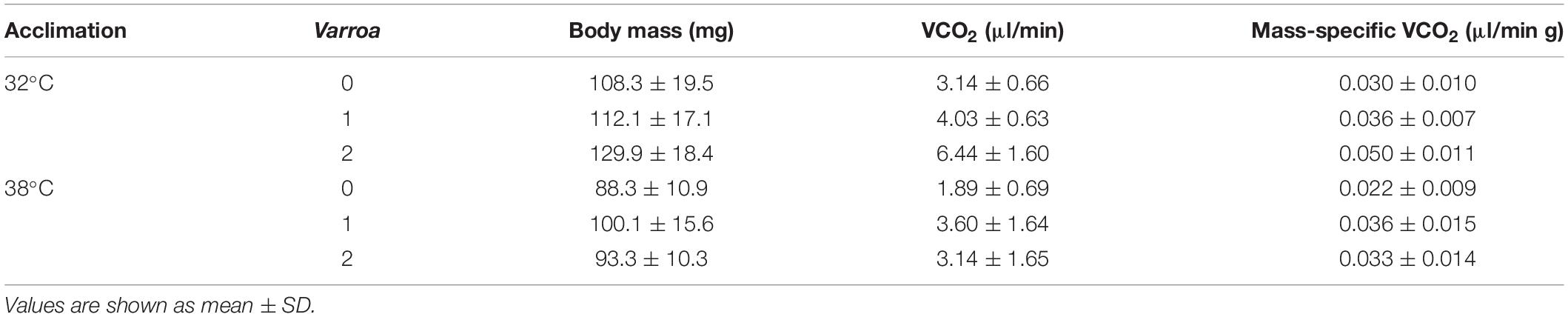
Table 2. Body mass and metabolic rate in honeybees from two thermal acclimation treatments subjected to different levels of parasitism (n = 10 for each group).
For logistic reasons, this experiment is constrained to acute responses to parasitism, acclimation to constant temperatures and we could not determine the outcome of the heat stress in mite’s survival, which is crucial to assess the feasibility of heat treatment to control Varroa infestation. TDT curves have been employed to develop thermal treatments for pest control (Tang et al., 2007), and the available information in the literature suggests that this is a realistic possibility (Table 3). Heat treatments result in high Varroa mortality, hence TDT curves can be employed to find optimal combinations of temperature and exposure times to control Varroa and other pathogens as long as the heat treatment does not negatively impact the honeybees (Figure 4; Rosenkranz, 1987; Goras et al., 2015; Bičík et al., 2016). Interestingly, despite the absence of standardized protocols across studies, multiple confounding factors and the uncertainty associated with many of the reported values (Table 3), published estimates of heat tolerance in Varroa vary in accordance with the framework employed here (Figure 4; Komissar, 1985; Kablau et al., 2019). A regression controlling for mortality as a factor (50% vs. 80–100% mortality) results in a CTmax = 52.4°C and z = 4.19°C, which suggests that Varroa can tolerate heat stress for longer than the honeybees in our study (Figure 2). This is not entirely surprising, however, because TDT curves reported here seem to underestimate heat tolerance of bees in the colony with access to water, food and shelter (see above), and most of the studies that we reviewed reported limited to negligible impact on brood and adult honeybees during thermal treatment (but see Harbo, 2000). Interestingly, higher honeybee mortality was generally observed during prolonged exposure to less extreme temperatures (48 to 76 h, see Harbo, 2000; Tabor and Ambrose, 2001), whereas treatments with an acute exposure to temperatures 42°C were generally less problematic for the bees. Consequently, our analyses strongly suggest that heat treatment may provide a viable solution to control Varroa and mitigate their impact on honeybees’ populations and the ecosystem services that they provide. Thus, more detailed studies of thermal tolerance in both honeybees and Varroa within the hives are necessary for a characterization of TDT curves under more realistic conditions and to design effective management strategies to deal with parasite.
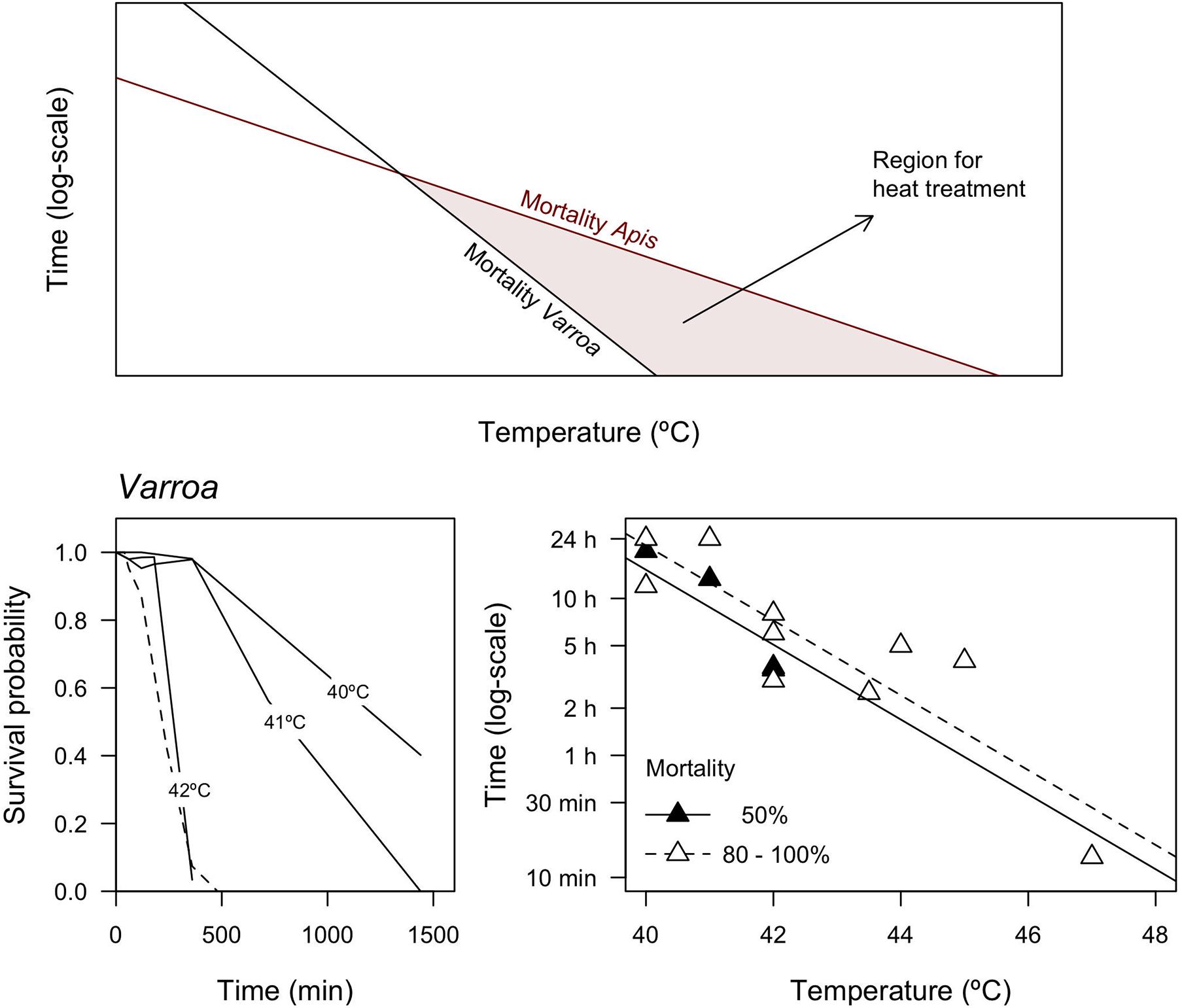
Figure 4. Heat treatment based on TDT curves of the honeybee and Varroa (based on Tang et al., 2007), applicable where the thermal tolerance of bees is higher than that of Varroa. Survival curves and mortality data from the literature suggest that thermal mortality in Varroa complies with expectations from TDT curves. Mortality of 50% was interpolated from survival curves, continuous, and dotted lines represent different studies (see Table 3).
Data Availability Statement
The raw data supporting the conclusions of this article will be made available by the authors, without undue reservation.
Author Contributions
PA-S maintained the honeybee colonies, carried out the assays, analyzed the data, and wrote the first draft of the manuscript. GR-C assisted with the heat tolerance assays and compilation of the data. ER and FB assisted with analyses and contributed to the final draft. All authors were involved in the design of the study.
Funding
This work was partly funded by FONDECYT 1170017 to ER and 1190007 to FB, and Fundación para la Innovación Agraria (FIA) PYT-2018-0058.
Conflict of Interest
The authors declare that the research was conducted in the absence of any commercial or financial relationships that could be construed as a potential conflict of interest.
Acknowledgments
We are grateful to all the team of Ecophysiology Laboratory in the University for all the support and help, and CONICYT PIA/BASAL FB0002 and to colleagues from CAPES assistance during assays.
Supplementary Material
The Supplementary Material for this article can be found online at: https://www.frontiersin.org/articles/10.3389/fevo.2021.656504/full#supplementary-material
Footnotes
References
Aldea, P., and Bozinovic, F. (2020). The energetic and survival costs of Varroa parasitism in honeybees. Apidologie 51, 997–1005. doi: 10.1007/s13592-020-00777-y
Annoscia, D., Del Piccolo, F., and Nazzi, F. (2012). How does the mite Varroa destructor kill the honeybee Apis mellifera? Alteration of cuticular hydrocarbons and water loss in infested honeybees. J. Ins. Phys. 58, 1548–1555. doi: 10.1016/j.jinsphys.2012.09.008
Becher, M., Scharpenberg, H., and Moritz, R. (2009). Pupal developmental temperature and behavioral specialization of honeybee workers (Apis mellifera L.). J. Comp. Physiol. A 195, 673–679. doi: 10.1007/s00359-009-0442-7
Bičík, V., Vagera, J., and Sádovská, H. (2016). The effectiveness of thermotherapy in the elimination of Varroa destructor. Acta Musei Sil. Sci. Natur. 65, 263–269. doi: 10.1515/cszma-2016-0032
Castañeda, L. E., Rezende, E. L., and Santos, M. (2015). Heat tolerance in Drosophila subobscura along a latitudinal gradient: contrasting patterns between plastic and genetic responses. Evolution 69, 2721–2734. doi: 10.1111/evo.12757
Cornelissen, B., Neumann, P., and Schweiger, O. (2019). Global warming promotes biological invasion of a honey bee pest. Glob. Change Biol. 25, 3642–3655. doi: 10.1111/gcb.14791
Crailsheim, K., Brodschneider, R., Aupinel, P., Behrens, D., Genersch, E., Vollmann, J., et al. (2012). “Standard methods for artificial rearing of Apis mellifera larvae,” in The COLOSS BEEBOOK: Standard Methods for Apis Mellifera Research, Vol. I, eds V. Dietemann, J. D. Ellis, and P. Neumann (New York, NY: Taylor & Francis). doi: 10.3896/IBRA.1.52.1.05
Dietemann, V., Nazzi, F., Martin, S., Anderson, D., Locke, B., Delaplane, K., et al. (2013). “Standard methods for Varroa research,” in The COLOSS BEEBOOK: Standard Methods for Apis Mellifera Pest and Pathogen Research, Vol. II, eds V. Dietemann, J. D. Ellis, and P. Neumann (New York, NY: Taylor & Francis). doi: 10.3896/IBRA.1.52.1.09
Emsen, B., Hamiduzzaman, M., Goodwin, P., and Guzman-Novoa, E. (2015). Lower virus infections in Varroa destructor-infested and uninfested brood and adult honey bees (Apis mellifera) of a low mite population growth colony compared to a high mite population growth colony. PLoS One 10:e0118885. doi: 10.1371/journal.pone.0118885
Erban, T., Sopko, B., Kadlikova, K., Talacko, P., and Haran, K. (2019). Varroa destructor parasitism has a greater effect on proteome changes than the deformed wing virus and activates TGF-β signaling pathways. Nat. Sci. Rep. 9:9400.
Evans, J., and Cook, S. (2018). Genetics and physiology of Varroa mites. Curr. Opin. Insect Sci. 26, 130–135. doi: 10.1016/j.cois.2018.02.005
Fries, I., Anton, I., and Rosenkranz, P. (2006). Survival of mite infested (Varroa destructor) honey bee (Apis mellifera) colonies in a Nordic climate. Apidologie 37, 564–570. doi: 10.1051/apido:2006031
Goras, G., Tananaki, C., Gounari, S., Dimou, M., Lazaridou, E., Karazafiris, E., et al. (2015). Hyperthermia-a non-chemical control strategy against varroa. J. Hel. Vet. Med. Soc. 66, 249–256. doi: 10.12681/jhvms.15869
Hamblin, A. L., Youngsteadt, E., López-Uribe, M. M., and Frank, S. D. (2017). Physiological thermal limits predict differential responses of bees to urban heat-island effects. Biol. Lett. 13:20170125. doi: 10.1098/rsbl.2017.0125
Harbo, J. (2000). Heating adult honey bees to remove Varroa jacobsoni. J. Apicult. Res. 39, 181–183. doi: 10.1080/00218839.2000.11101041
Hartfelder, K., Bitondi, M., Brent, C., GuidugliLazzarini, K., Simões, Z., Stabentheiner, A., et al. (2013). Standard methods for physiology and biochemistry research in Apis mellifera. J. Apicult. Res. 52, 1–48. doi: 10.3896/IBRA.1.52.1.06
Jones, J., Myerscough, M., Graham, S., and Oldroyd, B. (2004). Honey Bee Nest Thermoregulation: Diversity Promotes Stability. Available online at: www.sciencexpress.org (accessed June 24, 2004).
Kablau, A., Berg, S., Härtel, S., and Scheiner, R. (2019). Hyperthermia treatment can kill immature and adult Varroa destructor mites without reducing drone fertility. Apidologie 51, 307–315. doi: 10.1007/s13592-019-00715-7
Kang, Y., Blanco, K., Davis, T., Wang, Y., and DeGrandi-Hoffman, G. (2016). Disease dynamics of honeybees with Varroa destructor as parasite and virus vector. Math. Biosci. 275, 71–92. doi: 10.1016/j.mbs.2016.02.012
Klein, S., Cabirol, A., Devaud, J. M., Barron, A., and Lihoreau, M. (2017). Why bees are so vulnerable to environmental stressors. Trends Ecol. Evol. 32, 268–278. doi: 10.1016/j.tree.2016.12.009
Kovac, H., Kâfer, H., Stabentheiner, A., and Acosta, C. (2014). Metabolism and upper thermal limits of Apis mellifera carnica and A. m. ligustica. Apidologie 45, 664–677. doi: 10.1007/s13592-014-0284-3
Kovac, H., Stabentheiner, A., Hetz, S., Petz, M., and Crailsheim, K. (2007). Respiration of resting honeybees. J. Insect Physiol. 53, 1250–1261. doi: 10.1016/j.jinsphys.2007.06.019
Le Conte, Y., Arnold, G., and Desenfant, Ph. (1990). Influence of brood temperature and hygrometry variations on the development of the honey bee ectoparasite Varroa jacobsoni (Mesostigmata: Varroidae). Environ. Entomol. 19, 1780–1785. doi: 10.1093/ee/19.6.1780
Lee, K. V., Moon, R. D., Burkness, E. C., Hutchison, W. D., and Spivak, M. (2010). Practical sampling plans for Varroa destructor (Acari: Varroidae) in Apis mellifera (Hymenoptera: Apidae) colonies and apiaries. J. Econ. Entomol. 103, 1039–1050. doi: 10.1603/ec10037
Lighton, J. R. B., and Halsey, L. G. (2011). Flow-through respirometry applied to chamber systems: pros and cons, hints and tips. Compar. Biochem. Physiol. A 158, 265–275. doi: 10.1016/j.cbpa.2010.11.026
Maggi, M., Antúnez, K., Invernizzi, C., Aldea, P., Vargas, M., Negri, P., et al. (2016). Honeybee health in South America. Apidologie 47, 835–854.
Mandrioli, M. (2012). Someone like it hot? Effects of global warming on insect immunity and microbiota. ISJ 9, 58–63.
Maynard-Smith, J. (1957). Temperature tolerance and acclimatization in Drosophila subobscura. J. Exp. Biol. 34, 85–96. doi: 10.1242/jeb.34.1.85
Medrzycki, P., Sgolastra, F., Bortolotti, L., Bogo, G., Tosi, S., Padovani, E., et al. (2010). Influence of brood rearing temperature on honey bee development and susceptibility to poisoning by pesticides. J. Apicult. Res. 49, 52–59. doi: 10.3896/IBRA.1.49.1.07
Nazzi, F., and Le Conte, Y. (2016). Ecology of Varroa destructor, the major ectoparasite of the Western Honey Bee Apis mellifera. Annu. Rev. Entomol. 61, 417–432. doi: 10.1146/annurev-ento-010715-023731
Neumann, P., and Carreck, N. (2010). Honey bee colony losses. J. Apicult. Res. 49, 1–6. doi: 10.3896/IBRA.1.49.1.01
Ramsey, S., and van Engelsdorp, D. (2016). Varroa mites: beekeeper enemy number 1. Mellifera 16, 1–3.
Ramsey, S., Ochoa, R., Bauchan, G., Gulbronson, C., Mowery, J., Cohen, A., et al. (2019). Varroa destructor feeds primarily on honey bee fat body tissue and not hemolymph. Proc. Natl. Acad. Sci. U.S.A. 29, 1792–1801. doi: 10.1073/pnas.1818371116
Ratnieks, F., and Carreck, N. (2010). Clarity on honey bee collapse? Science 327, 152–153. doi: 10.1126/science.1185563
Requier, F., Antúnez, K., Morales, C., Aldea Sánchez, P., Castilhos, D., Garrido, P. M., et al. (2018). Trends in beekeeping and honey bee colony losses in Latin America. J. Apicult. Res. 57, 657–662. doi: 10.1080/00218839.2018.1494919
Rezende, E. L., Bozinovic, F., Szilágyi, A., and Santos, M. (2020). Predicting temperature mortality and selection in natural Drosophila populations. Science 369, 1242–1245. doi: 10.1126/science.aba9287
Rezende, E. L., Castañeda, L. E., and Santos, M. (2014). Tolerance landscapes in thermal ecology. Funct. Ecol. 28, 799–809. doi: 10.1111/1365-2435.12268
Rezende, E. L., Tejedo, M., and Santos, M. (2011). Estimating the adaptive potential of critical thermal limits: methodological problems and evolutionary implications. Funct. Ecol. 25, 111–121. doi: 10.1111/j.1365-2435.2010.01778.x
Richards, E. H., Jones, B., and Bowman, A. (2011). Salivary secretions from the honeybee mite, Varroa destructor: effects on insect haemocytes and preliminary biochemical characterization. Parasitology 138, 602–608. doi: 10.1017/s0031182011000072
Riveros, G., Arismendi, N., Zapata, N., Evans, D., Pérez, I., Aldea, P., et al. (2019). Occurrence, prevalence and viral load of deformed wing virus variants in Apis mellifera colonies in Chile. J. Apicult. Res. 59, 63–68. doi: 10.1080/00218839.2019.1670993
Robar, N., Murray, D., and Burness, G. (2011). Effects of parasites on host energy expenditure: the resting metabolic rate stalemate. Can. J. Zool. 89, 1146–1155. doi: 10.1139/z11-084
Rosenkranz, P. (1987). Temperature treatment of sealed worker brood as a method of controlling varroatosis. Apidologie 18, 385–388.
Rosenkranz, P., Aumeier, P., and Ziegelmann, B. (2010). Biology and control of Varroa destructor. J. Inver. Pathol. 103, 96–119.
Schäfer, O. M., Ritter, W., Pettis, J. S., and Neumann, P. (2010). Winter losses of honeybee colonies (Hymenoptera: Apidae): The role of Infestations with Aethina tumida (Coleoptera: Nitidulidae) and Varroa destructor (Parasitiformes: Varroidae). J. Econ. Entomol. 103, 10–16. doi: 10.1603/ec09233
Schmidt-Hempfel, P. (2008). Parasite immune evasion: a momentous molecular war. Trends Ecol. Evol. 23, 318–326. doi: 10.1016/j.tree.2008.02.011
Schmidt-Hempfel, P. (2009). Immune defence, parasite evasion strategies and their relevance for “macroscopic phenomena” such as virulence. Phil. Trans. R. Soc. B. 364, 85–98. doi: 10.1098/rstb.2008.0157
Tabor, K. L., and Ambrose, J. T. (2001). The use of heat treatment for control of the honey bee mite, Varroa destructor. Am. Bee J. 141, 733–736.
Tan, K., Radloff, S. E., Yusheng, Y., Yiqiu, L., Danyin, Z., and Neumann, P. (2005). Heat-balling wasp by honeybees. Naturwissenschaften 92, 492–495. doi: 10.1007/s00114-005-0026-5
Tang, J., Mitcham, E., Wang, E., and Lurie, S. (2007). Heat Treatment for Postharvest Pest Control. Trowbridge: Cromwell Press, xiv+349.
Tautz, J., Maier, S., Groh, C., Rössler, W., and Brockmann, A. (2003). Behavioral performance in adult honey bees is influenced by the temperature experienced during their pupal development. Proc. Natl. Acad. Sci. U.S.A. 100, 7343–7347. doi: 10.1073/pnas.1232346100
Keywords: Apis mellifera, metabolic rate, survival, thermal tolerance, Varroa, varroosis
Citation: Aldea-Sánchez P, Ramírez-Cáceres GE, Rezende EL and Bozinovic F (2021) Heat Tolerance, Energetics, and Thermal Treatments of Honeybees Parasitized With Varroa. Front. Ecol. Evol. 9:656504. doi: 10.3389/fevo.2021.656504
Received: 20 January 2021; Accepted: 24 June 2021;
Published: 16 July 2021.
Edited by:
Vera Maria Fonseca Almeida-Val, National Institute of Amazonian Research (INPA), BrazilReviewed by:
Derek Campos, National Institute of Amazonian Research (INPA), BrazilMichael Lattorff, International Centre of Insect Physiology and Ecology (ICIPE), Kenya
Copyright © 2021 Aldea-Sánchez, Ramírez-Cáceres, Rezende and Bozinovic. This is an open-access article distributed under the terms of the Creative Commons Attribution License (CC BY). The use, distribution or reproduction in other forums is permitted, provided the original author(s) and the copyright owner(s) are credited and that the original publication in this journal is cited, in accordance with accepted academic practice. No use, distribution or reproduction is permitted which does not comply with these terms.
*Correspondence: Patricia Aldea-Sánchez, plaldea@uc.cl