Ca2 +/Calmodulin-NOS/NO-TNFs Pathway Hallmarks the Inflammation Response of Oyster During Aerial Exposure
- 1Center of Deep Sea Research, Institute of Oceanology, Chinese Academy of Sciences, Qingdao, China
- 2CAS Key Laboratory of Experimental Marine Biology, Institute of Oceanology, Chinese Academy of Sciences, Qingdao, China
- 3Liaoning Key Laboratory of Marine Animal Immunology and Disease Control, Dalian Ocean University, Dalian, China
- 4Laboratory of Marine Fisheries Science and Food Production Processes, Qingdao National Laboratory for Marine Science and Technology, Qingdao, China
Aerial exposure (emersion) due to the periodical ebb and flow of tides is a major stressor for intertidal organisms and a key environmental factor in shaping their local communities. Oysters are among the most emersion-tolerant mollusk species and can survive for several days under aerial exposure. Noticeably, overwhelming inflammation responses could occur during the emersion stress. However, mechanisms beneath the activation and modulation of emersion-induced inflammation response have remained largely unknown. Ca2+ is an important intracellular second messenger that plays indispensable roles in inflammation response by cooperation with calmodulin (CaM) genes. Here, we showed that intracellular Ca2+ accumulates rapidly in oyster hemocytes during emersion stress along with the changes in the protein levels of three CaM genes, which function as intracellular sensors of Ca2+. As downstream effector of Ca2+/CaM complex, nitric oxide synthase (NOS) activity in hemocytes was enhanced during the emersion stress, facilitating a greater production of nitrite oxide (NO). Augmentation of NO concentration was associated with the increased mRNA expression levels of two oyster cytokines (CgTNFs) during aerial exposure. The robust accumulation of cytokines and severe injury of tissues in oysters have been regarded as potential cause and marker of their death in prolonged emersion stress. Here, both the expression levels of CgTNFs and the tissue injuries of oysters were attenuated when Ca2+/CaM complex or NOS activity were repressed in vivo during the emersion stress. These findings indicate that Ca2+/CaM-NOS/NO-CgTNFs pathway is critically involved in the emersion-induced inflammation response in oysters and plays a role in the resistance against long-term aerial exposure.
Introduction
The intertidal region is characterized by drastic fluctuations of physical parameters, such as tidal level and temperature, which makes the intertidal zone one of the most physiologically challenging environments for life. However, many organisms are thriving in the intertidal zone having evolved strategies to cope with such harsh and dynamically changing conditions. Adaptations of intertidal organisms have drawn significant attention in the past years (Richards, 2011; Teixeira et al., 2013; Cross et al., 2014; Goncalves et al., 2017; Maynard et al., 2018).
Aerial exposure or emersion due to periodical ebb and flow of tides is a major stressor for intertidal organisms associated with water loss, osmotic stress, hypoxia, and food deprivation (Strachan et al., 2015; Zhang et al., 2015). Notwithstanding, some intertidal organisms, especially algae and sessile mollusks, are well adapted to the periodical emersion morphologically, behaviorally, or physiologically (Diederich et al., 2015; Strachan et al., 2015; Duan et al., 2016). For example, some mollusks could close their shells or adhere more tightly to the rocky substratum to reduce water loss during emersion while some metabolic and developmental processes are repressed simultaneously for energy conservation (Sokolova and Pörtner, 2001; Hand and Menze, 2007). Moreover, the expression of protective macromolecules such as heat shock proteins and antioxidants including superoxide dismutase and catalase could also be significantly up-regulated to improve the tolerance against emersion stress (Pöhlmann et al., 2011; Jeno and Brokordt, 2014; Duan et al., 2016; Zhang et al., 2016). Nevertheless, overwhelming oxidative stress and inflammation response resulted from long-term aerial exposure could lead to cell apoptosis and irreversible tissue damage (Duan et al., 2016; Xin et al., 2016). However, there is limited empirical evidence demonstrating the mechanism underlying the responses to emersion, especially with regard to the biological processes during early resistance and final succumbing to stress in intertidal invertebrates.
Oysters are a common intertidal species exposed to emersion and associated abiotic stressors in the intertidal zone due to their sessile lifestyle. Oysters are stress-tolerant and can survive for several days in emersion in intertidal zone (Dong et al., 2017). During air exposure, the anaerobic metabolism of oysters is rapidly induced and heat shock proteins, antioxidant proteins, and inhibitor of apoptosis proteins are robustly up-regulated contributing to the tolerance against emersion (Seaman, 1991; Zhang et al., 2016). Furthermore, multiple genes involved in intracellular Ca2+ signaling pathway and neuro-endocrine system could also be modulated in oysters at early stage of emersion (Zhang et al., 2015; Dong et al., 2017). With growing studies in their genetic information and biological responses during emersion, oysters are becoming a suitable model for studying the adaptation mechanisms and physiological regulations of intertidal organisms against aerial exposure stress (Zhang et al., 2012). Recently, two members in oyster interleukin-17 family (CgIL17-1, CgIL17-5), which are pioneer cytokines in host inflammation response, were found drastically accumulated in hemocytes at the late stage of stress. It was further demonstrated that up-regulation of CgIL17s could result in the expressional decrease of inhibitor of apoptosis transcripts and increase of DNA fragmentation factor subunit alpha transcripts, contributing to the damage of hepatopancreas proceeding the death of oysters (Xin et al., 2016). These findings indicate that control of inflammation might play an important role in the tolerance of oysters to emersion, but the molecular mechanisms underlying the activation and modulation of this inflammatory response are not yet understood.
Calmodulin (CaM) is an important class of Ca2+-binding proteins involved in the Ca2+-mediated signaling pathway in both invertebrates and vertebrates, including mollusks (Hoeflich and Ikura, 2002; Han et al., 2020). Upon binding with Ca2+, CaMs could interact with numerous proteins to activate or inactivate them (Finn and Forsén, 1995). As a consequence, a variety of biological activities including cytokine expression and cell apoptosis could be switched on or off (Xu et al., 2011; Racioppi et al., 2012; Berchtold and Villalobo, 2014). Earlier studies showed that protein level of three CaMs proteins (CgCaM20234, accession number of EKC20234, CgCaM24243, accession number of EKC24243 and CgCaM24247, accession number of EKC24247) was markedly suppressed in gill of oysters during air exposure, indicating their involvement in host response to emersion stress (Zhang et al., 2015). Moreover, activity of nitric oxide synthase (NOS) could be promoted by Ca2+/CaMs complex in mammals and lead to a greater production of nitrite oxide (NO) (Abu-Soud et al., 1994; Michel et al., 1997; He et al., 2015). As demonstrated, NO could regulate the expression of several cytokines including tumor necrosis factors (TNFs) and accumulated during environmental stress, suggesting the potential role of Ca2+/CgCaMs complex in the emersion-induced inflammation response of oysters (Jiang et al., 2013; Bogdan, 2015; Su et al., 2018). However, few studies have reported Ca2+/CaM pathway in oysters, let alone their roles in emersion stress. In the present study, oyster Crassostrea gigas were subjected to emersion stress under the controlled laboratory conditions with the objectives to (1) survey the alternations of Ca2+ concentrations along with CgCaMs proteins during emersion stress, (2) assess the modulation role of Ca2+/CgCaMs complex in NOS/NO system and cytokine expression, and (3) depict the potential role of the Ca2+/CgCaMs pathway in oyster’s inflammation response against the emersion stress.
Materials and Methods
Oysters Preparation, Desiccation Stress, and Sample Collection
Adult oysters C. gigas (about 2-year old and 100–120 mm in shell length) were collected from a local aquaculture farm in Qingdao, China and acclimated in aerated and sterilized seawater for 2 weeks after they were sawed for a minor hole at shell close to adductor muscle for later injection. During the acclimation, mortality of oysters was monitored while very few individual was found succumbed.
A total of 190 oysters were subjected for aerial exposure (16°C, relative humidity 40%) using a sterilized box and placed in a constant temperature incubator (Shanghai Nanrong, SPX-160B). Fifteen oysters were sampled at 0, 1, 2, 3, 4, and 5 days of emersion to detect alternations of CgCaMs, CgTNF5109 and CgTNF6440 transcripts in hemocytes (hemocytes from five oysters were pulled together as one replicate). Hemocytes from another five oysters were also obtained at 0, 1, 3, and 5 days of emersion using acid citrate-dextrose anticoagulant agent (22 g L–1 sodium citrate, 8 g L–1 citric acid, 24.5 g L–1 glucose, pH 7.4) at a ratio of 8:1 and pulled together as one replicate to investigate cytoplasmic Ca2+/NO concentration and relative activity of NOS. The change of CgCaM protein levels was surveyed by Western blot using hemocytes of 10 oysters collected at 0, 1, 3, and 5 days of the aerial exposure. All assays except the Western blot were conducted simultaneously with three parallel replicates per group.
In vivo Inhibition of CaM and NOS
Calmidazolium chloride (CDZ, Santa Cruz) is a CaM antagonist that inhibits activities of CaM-dependent enzymes. L-NMMA (Beyotime), also known as NG-Hydroxy-L-arginine monoacetate, is a widely used inhibitor of NOS that competes with L-arginine. The sodium nitroferricyanide (III) dihydrate (SNP, Beyotime) is a donor for NO after in vivo breakdown. Inhibition of CaM and NOS was conducted here by adductor injection of these antagonists or inhibitors into oysters during aerial exposure. A functional rescue of NOS activity was also conducted in vivo by co-injection of CDZ and SNP. In detail, a total of 120 oysters were randomly divided into Aerial Exposure, Aerial Exposure+CDZ, Aerial Exposure+CDZ+SNP, and Aerial Exposure+L-NMMA groups. Oysters in each group were subjected to 2-day aerial exposure and injected with 100 μL diethyl pyrocarbonate (DEPC) water, 10 nM CDZ (in 100 μL DEPC water), 10 nM CDZ+5 mM SNP (in 100 μL DEPC water) or 0.5 mM L-NMMA (in 100 μL DEPC water) at 24 h of emersion, respectively. At the end of emersion stress, hemocytes from 15 random oysters (cells from five individual were pulled together as one replicate) in each group were collected with anticoagulant agent to detect NO concentration and NOS activity. Hemocytes from the remaining fifteen oysters (cells from five individual were pulled together as one replicate) were also obtained to survey transcripts of CgTNF5109 and CgTNF6440 by quantitative real-time PCR (qRT-PCR). All assays were conducted with three parallel replicates for each group.
A total of 20 oysters were employed for a continuous injection of CDZ and L-NMMA during aerial exposure to investigate morphological changes of hepatopancreas. Five of them were sampled immediately to demonstrate the healthy status of hepatopancreas before aerial exposure. The rest oysters were subjected to a 5-day emersion and injected with 100 μL DEPC water, 10 nM CDZ (in 100 μL DEPC water), or 0.5 mM L-NMMA (in 100 μL DEPC water) at 2, 3, and 4 days of emersion. The samples were collected at the fifth day of emersion and subjected to histological analysis along with samples obtained before the stress.
In vivo Knock-Down of CgCaM24243 by dsRNA-Mediated RNA Interference
Given the striking similarity of the coding regions among all CgCaM homologs, both the 5’-untranslated regions (UTR) and 3′-UTR of CgCaM24243, which was unique in oyster genome based on nucleotide BLAST result, were selected for dsRNA-mediated RNA interference. The UTR sequence was first retrieved according to the genome annotation information where sequence upstream the first exon was suggested as 5’-UTR and the sequence downstream the stop codon was putatively considered as 3′-UTR. Both sequences were then cloned using cDNA library from oyster hemocytes for verification and subjected to siDirect21 for siRNA prediction. The obtained sequences were then linked with T7 promoter (Supplementary Table S1) and subjected to in vitro dsRNA transcription using T7 RNA polymerase (Life technologies). After purification, the synthesized dsRNA was examined for integrity by electrophoresis and quantified by Nanodrop2000 (Life technologies). A cDNA fragment (657 bp) from pEGFP vector (Clontech) was used for the synthesis of control dsRNA (Xin et al., 2016).
A total of 60 oysters were randomly selected and divided in to Aerial Exposure group, Aerial Exposure+siCgCaM24243 group, and Aerial Exposure+siEGFP group. All oysters were then subjected to a 2-day aerial exposure and injected with 100 μL DEPC water or 100 μg dsRNA of CgCaM24243 or CgEGFP (in 100 μL DEPC water) at 24 h of emersion for RNA interference. At 48 h of emersion, hemocytes from five oysters in each group were sampled to survey the knock-down efficiency by Western blot. NOS activity and cytoplasmic NO concentration were surveyed from hemocytes of the remaining oysters while hemocytes from five oysters were polled together as one replicate and three replicates were used for each detection.
RNA Isolation, cDNA Synthesis, and Quantitative Real-Time PCR (qRT-PCR)
Total RNA extraction was conducted according to the manual of Trizol reagent (Life technologies) and subjected to cDNA synthesis using M-MLV Reverse Transcriptase (Promega). qRT-PCR for each gene was carried out with SYBR Premix Ex Taq II (Tli RNaseH Plus) kit (Takara) in ABI 7500 Real-Time PCR System (Applied Biosystems). Running program for the qRT-PCR was set in a two-step manner (denature at 95°C for 5 s, annealing and extension at 60°C for 34 s) with 40 cycles according to the manual while three replicates were employed for each trial. The relative mRNA expression level for genes was calculated with 2–ΔΔCt method. As demonstrated previously in either qRT-PCR assay or transcriptome sequencing, the expression patterns of oyster elongation factor gene remained comparatively stable across different tissues and during development or emersion stress were surveyed. Therefore, all detected transcripts were further normalized with CgEF1-α after the qRT-PCR assay (Du et al., 2013; Gong et al., 2019). All the primers employed in the present study were designed according to the genome information and listed in Supplementary Table S1. Products in the qRT-PCR assay were further verified by sequencing.
Western Blot and Densitometric Analysis
Western blot for CgCaM was conducted using methods described before (Xu et al., 2016). In brief, protein samples from oyster hemocytes were quantified by Bradford Protein Assay Kit (Beyotime) and subjected to SDS-PAGE to separate proteins. The proteins were then transferred to the nitrocellulose membrane and incubated with 3% BSA at room temperature for 1 h. The membrane was subsequently incubated with CALM3 polyclonal antibody (Abclonal) overnight at 4°C (dilution at 1:1000) for reaction with all CgCaMs. The HRP conjugated secondary antibody (Abclonal) was used to label target proteins, which were visualized by Thermo Scientific SuperSignal kit (Life technologies). β-Tubulin polyclonal antibody (Abcolnal) was used as the loading control in all Western blot assay. To quantify the alternations of CgCaM proteins in each group, densitometric analysis was conducted with ImageJ software2. Western blot results for β-tubulin were used here for normalization.
Quantification of Ca2+/NO Concentration and NOS Activity
Content of intracellular Ca2+ was determined using Calcium Colorimetric Assay (Sigma–Aldrich) following manufacturer’s instruction. Hemocyte pellet obtained without anticoagulant agent were first weighted using analytical balance (Sartorius BSA124S-CW, normalized with empty tubes) and then lyzed using lysis buffer. A volume of 100 μL cellular lysate obtained after centrifugation was mixed with the reaction buffer and incubated for 10 min in darkness. The absorbance was measured using a spectrophotometer at 575 nm. Ca2+ concentration was quantified according to standard curve and normalized by tissue mass.
Concentration of NO was detected by DAF-FM DA probe (Beyotime). Suspensions of oyster hemocytes were adjusted to 1 × 106cells/mL and incubated with 5 μM DAF-FM DA probe at room temperature for 30 min. After a wash with modified Leibovitz L-15 medium (Gibco) (Qiu et al., 2007), a total volume of 400 μL labeled cells were subjected to flow cytometry (FACS Arial II flow cytometer, BD Bioscience) for NO detection. A total of 10,000 oyster cells were tested for the DAF-FM DA fluorescence and the threshold for positive signals was set using cells free of probe incubation.
Activity of cellular NOS was determined using Nitric Oxide Synthase Assay Kit (Beyotime) according to the manufacturer’s instructions. Briefly, about 1 × 106 oyster hemocytes suspended in modified L-15 medium were incubated with L-Arginine, NADPH, and DAF-FM DA at room temperature for 30 min. After a wash with modified L-15 medium, 400 μL labeled cells were subjected to flow cytometry to detect relative NOS activity. Consistent with NO detection, a total of 10,000 oyster cells were tested for the fluorescence and the threshold for positive signals was set using hemocytes without probe incubation.
Histological Analysis of Hepatopancreas
Histological analysis of hepatopancreas was conducted with methods described before (Xin et al., 2016). In brief, hepatopancreas tissues collected after emersion were immediately fixed by 4% paraformaldehyde and embedded in paraffin after dehydration. The tissues were sectioned by a microtome (Leica) at 5 μm thickness, deparaffinised, rehydrated, and stained with hematoxylin and eosin (Beyotime).
Statistical Analysis
All data collected in the present study were given as means ± SD and assessed for normal distribution before subjected to one-way analysis of variance (ANOVA) with a multiple comparison (LSD method) using SPSS version 20 to verify the significant difference.
Results
Robust Response of Oyster Ca2+ and CgCaMs During Desiccation
Protein alternations of CgCaM20234, CgCaM24243, and CgCaM24247 were surveyed in oyster hemocytes and found significantly decreased during aerial exposure, reaching the lowest level at the third day of emersion (Figure 1A). The CgCaMs protein expression levels were partially recovered at the fifth day, but remained below the basal level. Meanwhile, cellular Ca2+ concentration increased rapidly during emersion stress and peaked at the third day of aerial exposure, reaching 2.02 mg/g (p < 0.05, Figure 1B). The Ca2+ concentration in hemocytes decreased afterward but remained elevated at 1.01 mg/g after 5 days of aerial exposure (Figure 1B).
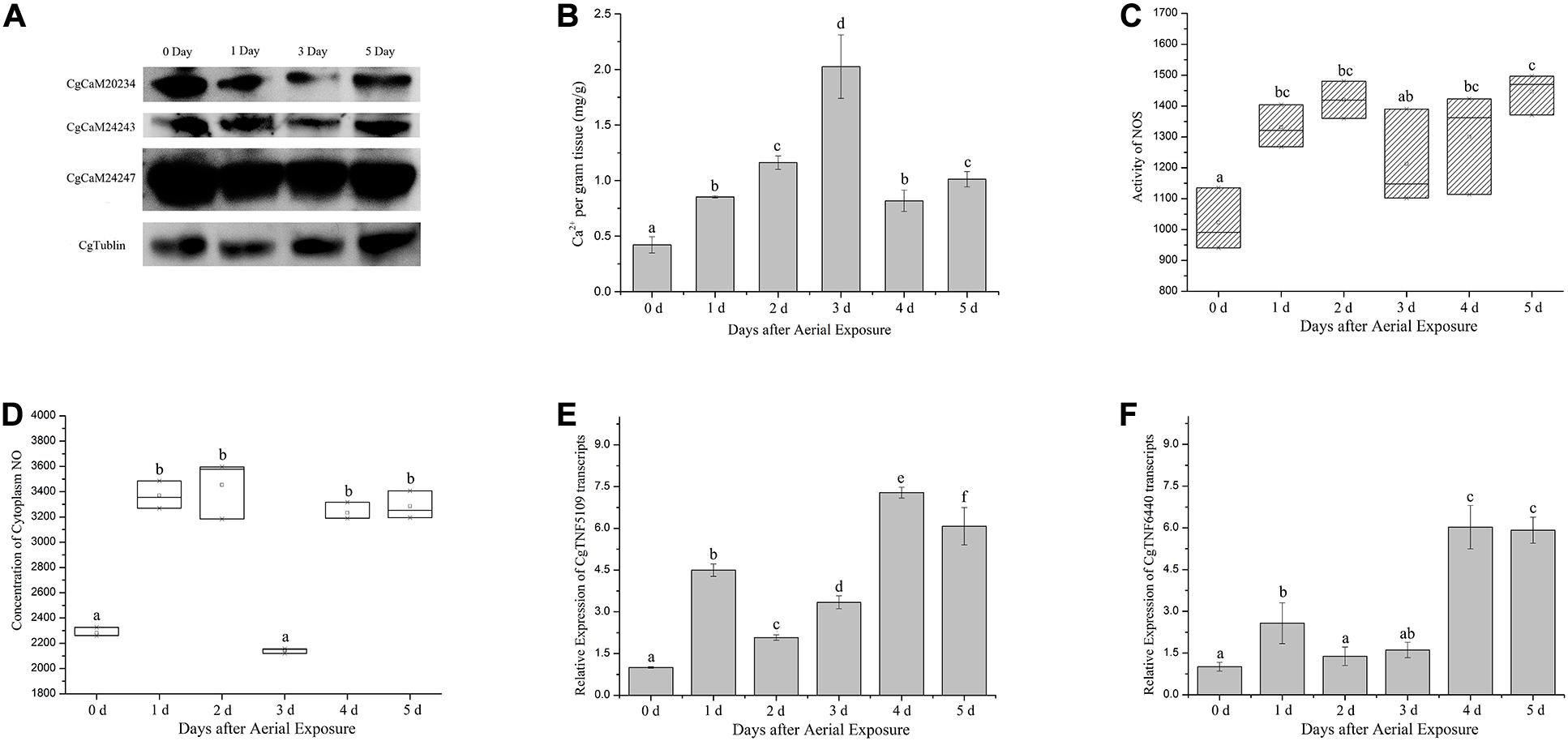
Figure 1. Physiological and molecular changes in oyster hemocytes during emersion. (A) Protein levels of three CgCaM proteins including CgCaM20234, CgCaM24243, and CgCaM24247 during aerial exposure. Robust decreases were observed in all studied CgCaMs at the first and third day of emersion while CgTublin remained unchanged. (B) Ca2+ concentration inside oyster hemocytes during emersion. A drastic increase of intracellular Ca2+ was observed starting from the first day of stress and peaked at the third day of emersion. (C,D) NOS activity and NO concentration in oyster hemocytes during the emersion. Activity of NOS increased significantly in oyster hemocytes during aerial exposure and decreased slightly at the third and fourth day (C). NO concentration inside oyster hemocytes increased significantly during the aerial exposure except for the third day when it dropped to the basal level (D). (E,F) Alternations of CgTNF5109 and CgTNF6440 transcripts during the emersion. CgTNF5109 transcripts were found up-regulated markedly in oyster hemocytes during the emersion, especially at the first, fourth, and fifth day of stress (E). CgTNF6440 transcripts were found accumulated drastically in oyster hemocytes at the fourth and fifth day of emersion (F). Significance was determined by one-way analysis of variance (ANOVA) and marked with letters (a,b,c, etc.) if p < 0.05.
The Up-Regulation of NOS/NO System and CgTNFs During Desiccation
The activity of NOS in oyster hemocytes increased significantly starting from the first day of emersion (1.30-fold of basal level at the first day, 1.39-fold at the second day, p < 0.05) and declined moderately on the third day of stress (1.18-fold of basal level, Figure 1C). NO concentration also increased markedly on the first day of aerial exposure (1.48-fold of basal level) and declined abruptly at the third day of stress, reaching the basal level (0.94-fold of basal level) (p < 0.05, Figure 1D). As the emersion stress continued, the NO concentration increased again, reaching 1.42-fold and 1.44-fold of basal level on the fourth and fifth day, respectively (p < 0.05, Figure 1D).
Transcript levels of two TNF-encoding genes (CgTNF5109 and CgTNF6440), which were found responsive to various stresses, were up-regulated on the first day of emersion to 4.50-fold and 2.57-fold of basal level, correspondingly (p < 0.05, Figures 1E,F). The mRNA levels of CgTNF genes slightly decreased after 2 and 3 days of emersion to 2.07-fold and 3.34-fold of basal level, correspondingly for CgTNF5109 (p < 0.05, Figure 1E), and to 1.38-fold and 1.60-fold of basal level, respectively, for CgTNF6440 (p < 0.05, Figure 1F). Later during the emersion, the transcription of the TNF-encoding genes increased again. The mRNA levels of CgTNF5109 reached 7.28-fold and 6.07-fold of the basal level at the fourth and fifth day, respectively (p < 0.05, Figure 1E). Similarly, CgTNF6440 increased to 6.02-fold of the basal level on the fourth day and 5.97-fold of the basal level on the fifth day (p < 0.05, Figure 1F).
Activation of Oyster NOS/NO-TNF Pathway by Ca2+/CaMs During Desiccation
Inhibition of CaM was then conducted in vivo during the emersion by injection of a CaM antagonist CDZ. Consequently, intracellular NOS activity and NO concentration decreased after CDZ injection to 0.59-fold and 0.50-fold of the basal level, respectively (p < 0.01, Figures 2A,B). When oysters were co-injected with CDZ and an NO donor SNP, the NO concentration inside hemocytes was partially restored to 0.76-fold of the basal level (p < 0.01, Figure 2B). When oysters were injected with an NOS inhibitor L-NMMA, the NO concentration dropped to 0.79-fold of the basal level (p < 0.01, Figure 2B).
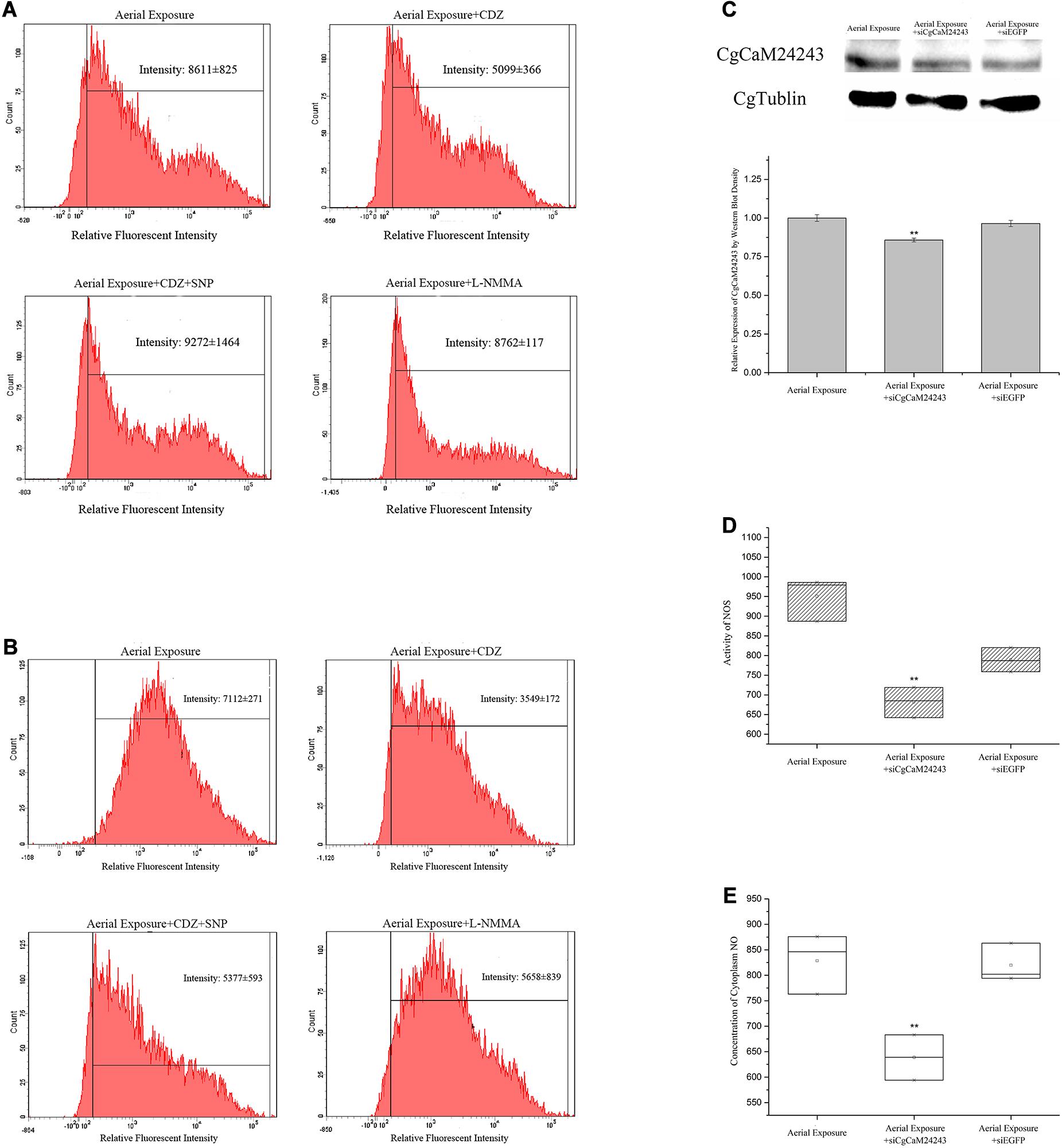
Figure 2. Changes in oyster NOS/NO system after functional inhibition of CaMs and NOS. (A,B) Alternations of NOS activity and NO concentration inside hemocytes of oyster injected with CDZ (Calmidazolium chloride, CaM antagonist), CDZ+SNP (NO donor), or L-NMMA (NOS inhibitor) during a 2-day emersion. Significant decrease of NOS activity (A) and NO concentration (B) was observed in Aerial exposure+CDZ group where CaMs were suggested to be repressed by CDZ. The decrease in NO concentration was rescued in Aerial exposure+CDZ+SNP group where NO donor was provided. NO concentration also decreased in Aerial exposure+L-NMMA group where NOS activity was suppressed by L-NMMA. (C) Known-down of CgCaM24243 gene by dsRNA injection. Protein level of CgCaM24243 dropped significantly in oyster hemocytes after injection of dsRNA during the aerial exposure. Comparatively, CgCaM24243 protein remained unchanged in Aerial Exposure+siEGFP group where oysters were injected with dsRNA of pEGFP vector. A densitometric analysis was conducted to facilitate the significance determination. (D,E) NOS activity and NO concentration after the in vivo known-down of CgCaM24243 gene. Both the NOS activity (D) and NO concentration (E) in hemocytes were found significantly decreased in Aerial Exposure+siCgCaM24243 group where dsRNA of CgCaM24243 was injected during emersion. Aerial Exposure+siEGFP group was used as negative control. Significance was determined by one-way analysis of variance (ANOVA) and marked with ** if p < 0.01.
Knock-down of CgCaM24243 by injecting dsRNA during emersion led to a decrease of the CgCaM24243 protein when compared with the air-exposed group that did not receive dsRNA injection or received an injection of a control dsRNA (Aerial Exposure+siEGFP group) (p < 0.01, Figure 2C). The NOS activity declined significantly to 0.86-fold of the control group after knock-down of CgCaM24243 (p < 0.01, Figure 2D). A significant decrease of NO concentration (to 0.77-fold of the control group) was also observed in knock-down assay of CgCaM24243 (p < 0.01, Figure 2E). Furthermore, mRNA levels of CgTNF5109 and CgTNF6440 were significantly down-regulated in response to the functional inhibition of CgCaMs and NOS. As shown in Figure 3A, transcripts of CgTNF5109 decreased to 0.64-fold of the basal level in Aerial Exposure+CDZ group (p < 0.05). When SNP was co-injected with CDZ, CgTNF5109 expression level returned to the same levels as seen in the air exposed group (Figure 3A). The transcripts of CgTNF5109 declined to 0.56-fold of basal level after suppression of NOS by L-NMMA (p < 0.05, Figure 3A). Similarly, transcripts of CgTNF6440 decreased to 0.51-fold of the levels found in the air exposed group in CDZ injection group and but remained unchanged when SNP was co-injected (p < 0.05, Figure 3A). When NOS activity was repressed by L-NMMA, CgTNF6440 expression level dropped to 0.63-fold of the levels seen in the air-exposed group (p < 0.05, Figure 3A).
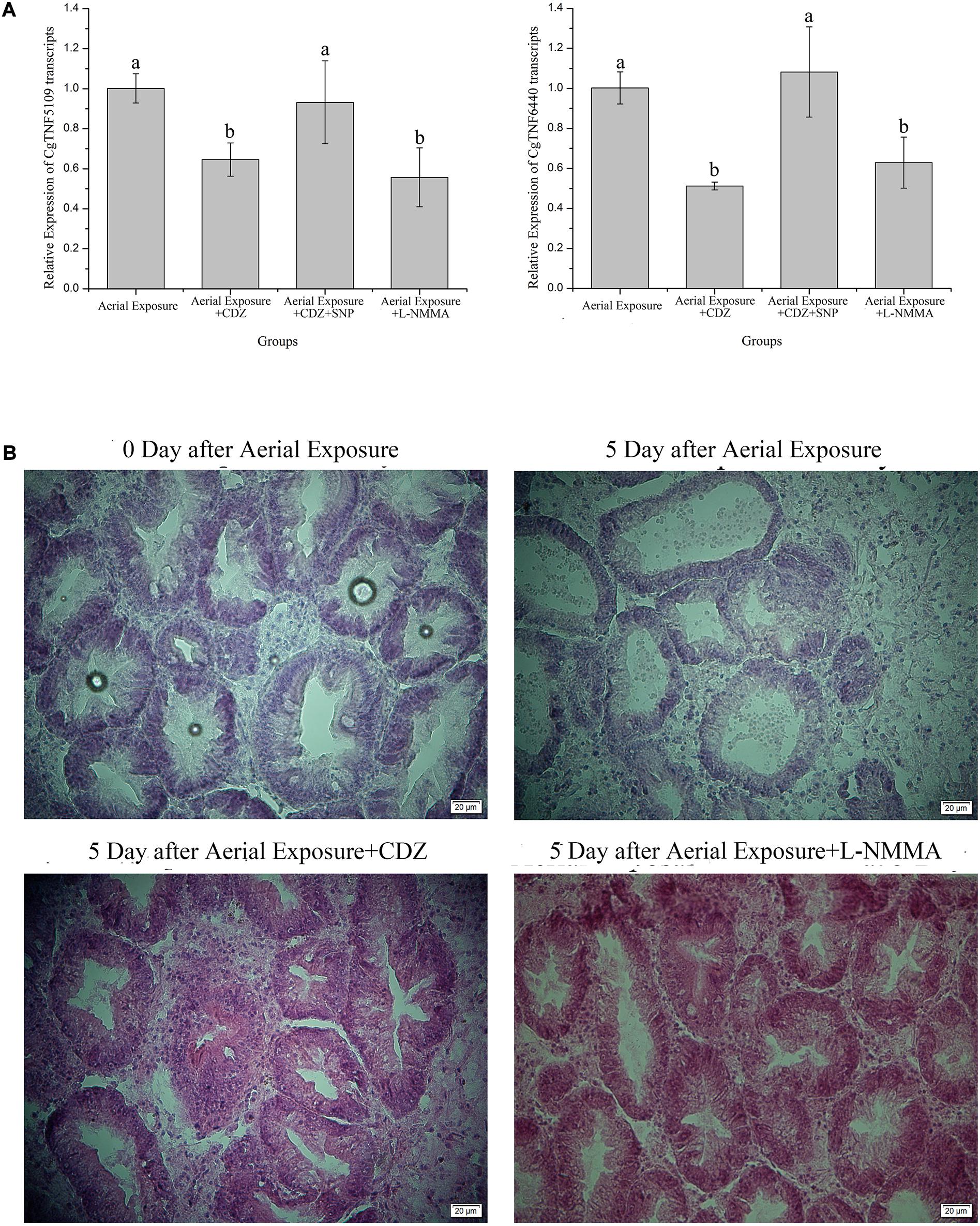
Figure 3. Alternations of CgTNFs transcripts and hepatopancreas morphology after functional inhibition of CaMs and NOS. (A) Expression levels of CgTNF5019 and CgTNF6440 in oyster hemocytes after functional inhibition of CaMs and NOS during emersion. Both genes were found decreased significantly when CaMs were suppressed by CDZ or when NOS activity was repressed by L-NMMA. Transcripts of CgTNFs regained to basal level when NO donor was supplemented during CaMs inhibition. Significance was determined by one-way analysis of variance (ANOVA) and marked with letters (a,b,c, etc.) if p < 0.05. (B) Hematoxylin and eosin staining of hepatopancreas after the functional inhibition of CaMs and NOS during emersion. As shown, digestive tubules of oyster hepatopancreas were pathologically expanded after a 5-day emersion while massive vacuolization was also observed in the epithelial cells. When CaMs and NOS were repressed during emersion by continuous injection of CDZ or L-NMMA, the pathological expansion of digestive tubules along with vacuoles of epithelial cells was rescued.
Tissue Injury Relieved After Repression of Ca2+/CgCaMs-NOS/NO Pathway
Histopathological changes of oyster hepatopancreas were detected after inhibiting oyster CaM and NOS. The digestive tubules of oyster hepatopancreas were pathologically expanded and massive vacuolization could be observed in the epithelial cells at the fifth day post desiccation, demonstrating severe injury of hepatopancreas (Figure 3B and Supplementary Figure S1). When oysters were continuously injected with CaM inhibitors during emersion, the histopathological injury was significantly relieved with less expanded cavity spaces (Figure 3B and Supplementary Figure S1). Suppression of the hepatopancreas damage in desiccation could also be observed in Aerial Exposure+L-NMMA group (compared with the air exposure only) in which NOS activity was inhibited (Figure 3B and Supplementary Figure S1).
Discussion
As a major stressor for organisms inhabiting the intertidal zone, aerial exposure (emersion) has been regarded as a decisive factor in their colony distribution by shaping their physiological and biochemical features (Hand and Menze, 2007). The oysters are a dominant mollusk in most intertidal region and known as an emersion-tolerant invertebrate as it could survive for several days under aerial exposure (Dong et al., 2017). How oysters respond to emersion stress is therefore noteworthy and could be informative in understanding the adaptation of intertidal organisms against aerial exposure. In a recent study, two members out of 10 IL-17 genes were found fast induced in transcription level in oyster hemocytes and lead to severe injury of hepatopancreas tissue afterward, suggesting the crucial role of inflammation response in oysters’ resistance against emersion (Xin et al., 2016). At the meantime, protein levels of three CgCaMs genes (CgCaM24247, CgCaM20234, and CgCaM24243) were down-regulated in oyster gills during emersion (Zhang et al., 2015). Given the modulatory role of CaMs in either cytokine expression or cell apoptosis, it is a necessity to ascertain the potential role of CgCaMs in inflammation response of oysters. Hemocytes are the main immunocytes of oysters responsible for cytokine expression and secretion (Ottaviani, 2010; Wang et al., 2017; Gerdol et al., 2018). Therefore, the expression patterns of these three CgCaM genes during emersion were surveyed exclusively in oyster hemocytes. Consistent with previous findings, all studied CgCaMs were down-regulated in oyster hemocytes during aerial exposure. Notwithstanding, Ca2+ concentration was found altered in an opposite pattern of CgCaMs during emersion. As an important class of intracellular Ca2+ sensors, the proper function of CaMs relies greatly on the binding of cytoplasmic Ca2+ (Hoeflich and Ikura, 2002; Clapham, 2007). The paradox in Ca2+ concentration and CgCaMs protein level brought forth questions about the potential influences of Ca2+/CgCaM complex in the inflammation response of oysters against emersion.
As an important signal transducer in multicellular organisms, a diversity of proteins could be regulated by Ca2+/CaM complex, including myosin-light-chain kinase, phosphorylase kinase, serine/threonine-protein phosphatase, calcium/CaM-dependent protein kinase I, NOS, and adenylate cyclase (Chin and Means, 2000; Vetter and Leclerc, 2003). Among these genes, NOS is of interest due to its role in inflammation response and stress response of multiple organisms (Bogdan, 2001; Tripathi et al., 2007). What is more, recent studies in some mollusks also demonstrated the modulation role of Ca2+/CaMs complex in NOS activation (Bodnárová et al., 2005). Thus, the alternations of NOS activity were surveyed in oyster hemocytes during emersion stress. It turned out that NOS activity increased significantly in oyster hemocytes starting from the first day of stress. As products of NOS, NO concentration inside hemocytes also increased drastically during the stress, confirming the alternation of NOS activity while suggesting the interaction between Ca2+/CgCaMs complex and NOS in oysters. To further verify the interaction between Ca2+/CgCaMs and NOS/NO system, functional inhibitions of CaMs and NOS were conducted in vivo during aerial exposure. As expected, NOS activity and NO concentration decreased significantly after injecting antagonists or dsRNA of CgCaMs, certificating the involvement of Ca2+/CaMs-NOS/NO pathway in the stress response of oysters against emersion. Moreover, the augment of NOS/NO system has also indicated a functional activation of Ca2+/CgCaMs complex despite of the paradox in Ca2+ concentration and CgCaMs protein level. It is also worthy to notice that several types of NOS (iNOS, eNOS, and nNOS) were encoded by vertebrates while only one NOS-encoding gene is identified in oyster genome (Alderton et al., 2001; Zhang et al., 2012). The present results demonstrated that oyster NOS gene could retain a primitive form in comparison with its vertebrate homologs, reconfirming our previous findings (Jiang et al., 2016). It was therefore interesting to figure out whether oyster cytokines could also be modulated by Ca2+/CaMs-NOS/NO pathway during desiccation-induced stress response.
Being a highly diffusible gas and ubiquitous bioactive molecule, NO could modulate the expression of multiple cytokines including TNF, interferon, and ILs (Bogdan, 2015). Previous study has shown that transcripts of two CgIL-17 genes could be induced in oyster hemocytes due to the elevated glucose concentration in serum during emersion (Xin et al., 2016). Besides IL17 family members, multiple TNF-encoding genes have also been identified in oysters and participating in the inflammation response against biotic and abiotic stress (Sun et al., 2014; Gao et al., 2015; Chen et al., 2016). Therefore, expression alternations of two representative CgTNF genes (CgTNF5109 and CgTNF6440) were characterized during emersion. Consequently, both CgTNFs were up-regulated during aerial exposure. Meanwhile, transcripts of CgTNF5109 and CgTNF6440 were significantly suppressed when CgCaMs or NOS were functionally inhibited. These results collectively demonstrated the involvement of Ca2+/CaM-NOS/NO pathway in the transcriptional promotion of CgTNFs during emersion stress. As the main immunocytes, secreted cytokines from hemocytes could endow a ubiquitous promotion of inflammation response in different tissues or organs (Gerdol et al., 2018). It was demonstrated that tissue injury oysters could be recused markedly when CgIL-17s were suppressed, depicting the crucial role of inflammatory cytokines in the resistance of oysters against prolonged emersion stress (Xin et al., 2016; Dong et al., 2017). Since CgTNFs could be transcriptionally promoted by Ca2+/CaMs-NOS/NO pathway during emersion, it is therefore obligatory to know whether above pathway could result in pathological injury in oysters during emersion. By taking the hepatopancreas tissue as example, it was found that pathological expansion and massive vacuolization observed during prolonged aerial exposure could be greatly reduced after continuous inhibition of CaMs and NOS during emersion. This finding reconfirmed aforesaid speculation that cytokine-mediated inflammation response could influence the resistance of oysters against long-term emersion stress.
As a highly integrated immunological process, inflammation response is mainly mediated by cytokines and meant to protect the organisms from either biotic or abiotic stress (Barton, 2008). The inflammation response, however, could be harmful to the host if out of control (Lassmann, 2008). As demonstrated, the robust accumulation of two CgIL-17 transcripts during emersion was due to the elevated glucose concentration in hemolymph, which was induced by glycogenolysis in a glycogen synthase kinase-3β-dependant way (Xin et al., 2016). Though NOS/NO-CgTNFs pathway was found continuously promoted in oyster hemocytes in a Ca2+/CgCaM-dependent way, how Ca2+ and CgCaMs were modulated remained less investigated here. Notwithstanding, it has been demonstrated that concentration of intracellular Ca2+ could be promoted as by-products of glucose uptake (Berna et al., 2002). Meanwhile, a rapid increase of glucose uptake was observed in either endothelial cells under hypoxia stress or in oysters under desiccation stress (Yamada et al., 2000; Wang et al., 2016). Therefore, the augment of intracellular Ca2+ is supposed to be resulted from the promotion of glucose uptake in oyster hemocytes. Given the great energy demand of oysters in dealing with stress, it was suggested that augment of intracellular Ca2+ could be unavoidable during prolonged emersion, resulting in the continuous promotion of Ca2+/CgCaM-NOS/NO-CgTNFs pathway. Moreover, considering that NO production could be promoted by CgTNFs reciprocally, the continuous promotion of Ca2+/CgCaM-mediated inflammatory pathway seems to be irreversible in long-term emersion stress, compromising the homeostasis of inflammation response (Zheng et al., 2020). Interestingly, a significant repression of CgCaMs proteins was observed starting from the first day of emersion before regaining at the late-stage of stress. Although the modulation mechanism of CgCaMs remained unknown, it is speculated that the expressional decrease of CgCaMs could be a protection against the overwhelmingly promoted inflammation response counteracting increase of intracellular Ca2+. Lastly, there are also some limitations in our study. For example, all the oysters were pre-drilled before the experiment and the emersion stress was conducted in laboratory condition with consistent temperature and humidity. The physiological and molecular response of oysters against emersion could differentiate from the stress responses in the wild since the energy consumption and gene expression might be allocated for shell repair (Hüning et al., 2016). Nevertheless, results of the present study are still informative in understanding the inflammation response of oyster against aerial exposure where Ca2+/CgCaM-NOS/NO-CgTNFs pathway is suggested as an important hallmark.
Data Availability Statement
The original contributions presented in the study are included in the article/Supplementary Material. Further inquiries can be directed to the corresponding author/s.
Ethics Statement
This animal study was reviewed and approved by the Experimental Animal Ethics Committee, Institute of Oceanology, Chinese Academy of Sciences, China.
Author Contributions
HC, LX, and LinW carried out the experiment and data analysis, participated in the design of the study, and drafted the manuscript. HZ, LinW, RL, and HW participated in the design of the study and discussed the results. LingW and LS conceived of the study, coordinated the experiment, and helped draft the manuscript. XQ helped review the manuscript. All authors gave final approval for publication.
Funding
This research was supported by National Key R&D Program (2018YFD0900606), grants (Nos. 41961124009 and U1706204) from the National Science Foundation of China, earmarked fund (CARS-49) from Modern Agro-industry Technology Research System, the Fund for Outstanding Talents and Innovative Team of Agricultural Scientific Research, Key R&D Program of Liaoning Province (2017203001 to LingW), Aoshan Talents Cultivation Program Supported by Qingdao National Laboratory for Marine Science and Technology (No. 2017ASTCP-OS13), the Distinguished Professor of Liaoning (to LS), and the Research Foundation for Talented Scholars in Dalian Ocean University (to LingW).
Conflict of Interest
The authors declare that the research was conducted in the absence of any commercial or financial relationships that could be construed as a potential conflict of interest.
Acknowledgments
The authors are grateful to all the laboratory members for technical advice and helpful discussions.
Supplementary Material
The Supplementary Material for this article can be found online at: https://www.frontiersin.org/articles/10.3389/fmars.2020.603825/full#supplementary-material
Supplementary Figure 1 | Hepatopancreas morphology after functional inhibition of CaMs and NOS. (A) HE staining of hepatopancreas from oysters before emersion stress (N = 3). (B) HE staining of hepatopancreas from oysters after a 5-day emersion stress (N = 3). (C) HE staining of hepatopancreas from oysters after functional inhibition of CaMs during the emersion stress (N = 3). (D) HE staining of hepatopancreas from oysters after functional inhibition of NOS during the emersion stress (N = 3).
Supplementary Table 1 | Primers used in this study.
Footnotes
References
Abu-Soud, H. M., Yoho, L. L., and Stuehr, D. J. (1994). Calmodulin controls neuronal nitric-oxide synthase by a dual mechanism. Activation of intra- and interdomain electron transfer. J. Biol. Chem. 269, 32047–32050.
Alderton, W. K., Cooper, C. E., and Knowles, R. G. (2001). Nitric oxide synthases: structure, function and inhibition. Biochem. J. 357, 593–615. doi: 10.1042/bj3570593
Barton, G. M. (2008). A calculated response: control of inflammation by the innate immune system. J. Clin. Invest. 118, 413–420. doi: 10.1172/JCI34431
Berchtold, M. W., and Villalobo, A. (2014). The many faces of calmodulin in cell proliferation, programmed cell death, autophagy, and cancer. Biochim. Biophys. Acta Mol. Cell Res. 1843, 398–435. doi: 10.1016/j.bbamcr.2013.10.021
Berna, N., Arnould, T., Remacle, J., and Michiels, C. (2002). Hypoxia-induced increase in intracellular calcium concentration in endothelial cells: Role of the Na+-glucose cotransporter. J. Cell. Biochem. 84, 115–131. doi: 10.1002/jcb.1271
Bodnárová, M., Martásek, P., and Moroz, L. L. (2005). Calcium/calmodulin-dependent nitric oxide synthase activity in the CNS of Aplysia californica: Biochemical characterization and link to cGMP pathways. J.Inorg. Biochem. 99, 922–928. doi: 10.1016/j.jinorgbio.2005.01.012
Bogdan, C. (2001). Nitric oxide and the immune response. Nat. Immunol. 2, 907–916. doi: 10.1038/ni1001-907
Bogdan, C. (2015). Nitric oxide synthase in innate and adaptive immunity: an update. Trends Immunol. 36, 161–178. doi: 10.1016/j.it.2015.01.003
Chen, H., Jiang, S., Wang, L., Wang, L., Wang, H., Qiu, L., et al. (2016). Cgi-miR-92d indirectly regulates TNF expression by targeting CDS region of lipopolysaccharide-induced TNF-alpha factor 3 (CgLITAF3) in oyster Crassostrea gigas. Fish & Shellfish Immunology 55, 577–584. doi: 10.1016/j.fsi.2016.06.036
Chin, D., and Means, A. R. (2000). Calmodulin: a prototypical calcium sensor. Trends Cell Biol. 10, 322–328. doi: 10.1016/S0962-8924(00)01800-6
Cross, I., Merlo, M. A., Rodriguez, M. E., Portela-Bens, S., and Rebordinos, L. (2014). Adaptation to abiotic stress in the oyster Crassostrea angulata relays on genetic polymorphisms. Fish Shellfish Immunol. 41, 618–624. doi: 10.1016/j.fsi.2014.10.011
Diederich, C. M., Bashevkin, S. M., Chaparro, O. R., and Pechenik, J. A. (2015). Desiccation tolerance and lifting behavior in Crepidula fornicata (Gastropoda). Mar. Ecol. Prog. Ser. 528, 235–243. doi: 10.3354/meps11284
Dong, W., Liu, Z., Qiu, L., Wang, W., Song, X., Wang, X., et al. (2017). The modulation role of serotonin in Pacific oyster Crassostrea gigas in response to air exposure. Fish Shellfish Immunol. 62, 341–348. doi: 10.1016/j.fsi.2017.01.043
Du, Y., Zhang, L., Xu, F., Huang, B., Zhang, G., and Li, L. (2013). Validation of housekeeping genes as internal controls for studying gene expression during Pacific oyster (Crassostrea gigas) development by quantitative real-time PCR. Fish Shellfish Immunol. 34, 939–945. doi: 10.1016/j.fsi.2012.12.007
Duan, Y., Zhang, Y., Dong, H., and Zhang, J. (2016). Effect of desiccation on oxidative stress and antioxidant response of the black tiger shrimp Penaeus monodon. Fish Shellfish Immunol. 58, 10–17. doi: 10.1016/j.fsi.2016.09.004
Finn, B. E., and Forsén, S. (1995). The evolving model of calmodulin structure, function and activation. Structure 3, 7–11. doi: 10.1016/S0969-2126(01)00130-7
Gao, D., Qiu, L., Gao, Q., Hou, Z., Wang, L., and Song, L. (2015). Repertoire and evolution of TNF superfamily in Crassostrea gigas: implications for expansion and diversification of this superfamily in Mollusca. Dev. Comp. Immunol. 51, 251–260. doi: 10.1016/j.dci.2015.04.006
Gerdol, M., Gomez-Chiarri, M., Castillo, M. G., Figueras, A., Fiorito, G., Moreira, R., et al. (2018). “Immunity in molluscs: recognition and effector mechanisms, with a focus on bivalvia,” in Advances in Comparative Immunology, ed. E. L. Cooper (Cham: Springer International Publishing), 225–341.
Goncalves, P., Jones, D. B., Thompson, E. L., Parker, L. M., Ross, P. M., and Raftos, D. A. (2017). Transcriptomic profiling of adaptive responses to ocean acidification. Mol. Ecol. 26, 5974–5988. doi: 10.1111/mec.14333
Gong, C., Liu, C., Li, H., Li, M., Liu, Z., Wang, W., et al. (2019). The transformation of energy metabolism and endoplasmic reticulum stress regulation in Pacific oyster Crassostrea gigas under air exposure. Inverteb. Survival J. 16, 72–83.
Han, Y., Tang, Y., Sun, S., Kim, T., Ju, K., Ri, S., et al. (2020). Modulatory function of calmodulin on phagocytosis and potential regulation mechanisms in the blood clam Tegillarca granosa. Dev. Comp. Immunol. [Epub ahead of print] doi: 10.1016/j.dci.2020.103910
Hand, S. C., and Menze, M. A. (2007). Desiccation stress. Encyclopaedia of tide pools and rocky shores. Berkeley: University of California Press, 173–177.
He, Y., Haque, M. M., Stuehr, D. J., and Lu, H. P. (2015). Single-molecule spectroscopy reveals how calmodulin activates NO synthase by controlling its conformational fluctuation dynamics. Proc. Natl. Acad. Sci. U.S.A. 112, 11835–11840. doi: 10.1073/pnas.1508829112
Hoeflich, K. P., and Ikura, M. (2002). Calmodulin in action: diversity in target recognition and activation mechanisms. Cell 108, 739–742. doi: 10.1016/S0092-8674(02)00682-7
Hüning, A. K., Lange, S. M., Ramesh, K., Jacob, D. E., Jackson, D. J., Panknin, U., et al. (2016). A shell regeneration assay to identify biomineralization candidate genes in mytilid mussels. Mar. Genomics 27, 57–67. doi: 10.1016/j.margen.2016.03.011
Jeno, K., and Brokordt, K. (2014). Nutritional status affects the capacity of the snail Concholepas concholepas to synthesize Hsp70 when exposed to stressors associated with tidal regimes in the intertidal zone. Mar. Biol. 161, 1039–1049. doi: 10.1007/s00227-014-2397-7
Jiang, Q., Liu, Z., Zhou, Z., Wang, L., Wang, L., Yue, F., et al. (2016). Transcriptional activation and translocation of ancient NOS during immune response. FASEB J. 30, 3527–3540. doi: 10.1096/fj.201500193RR
Jiang, Q., Zhou, Z., Wang, L., Shi, X., Wang, J., Yue, F., et al. (2013). The immunomodulation of inducible nitric oxide in scallop Chlamys farreri. Fish Shellfish Immunol. 34, 100–108. doi: 10.1016/j.fsi.2012.10.011
Lassmann, H. (2008). Mechanisms of inflammation induced tissue injury in multiple sclerosis. J. Neurol. Sci. 274, 45–47. doi: 10.1016/j.jns.2008.04.003
Maynard, A., Bible, J. M., Pespeni, M. H., Sanford, E., and Evans, T. G. (2018). Transcriptomic responses to extreme low salinity among locally adapted populations of Olympia oyster (Ostrea lurida). Mol. Ecol. 27, 4225–4240. doi: 10.1111/mec.14863
Michel, J. B., Feron, O., Sacks, D., and Michel, T. (1997). Reciprocal Regulation of Endothelial Nitric-oxide Synthase by Ca2+-calmodulin and caveolin. J. Biol. Chem. 272, 15583–15586. doi: 10.1074/jbc.272.25.15583
Ottaviani, E. (2010). Immunocyte: the invertebrate counterpart of the vertebrate macrophage. Inverteb. Survival J. 8, 1–4.
Pöhlmann, K., Koenigstein, S., Alter, K., Abele, D., and Held, C. (2011). Heat-shock response and antioxidant defense during air exposure in Patagonian shallow-water limpets from different climatic habitats. Cell Stress Chaperones 16, 621–632. doi: 10.1007/s12192-011-0272-8
Qiu, L., Song, L., Xu, W., Ni, D., and Yu, Y. (2007). Molecular cloning and expression of a Toll receptor gene homologue from Zhikong Scallop. Chlamys farreri. Fish Shellfish Immunol. 22, 451–466. doi: 10.1016/j.fsi.2006.05.003
Racioppi, L., Noeldner, P. K., Lin, F., Arvai, S., and Means, A. R. (2012). Calcium/calmodulin-dependent protein kinase kinase 2 regulates macrophage-mediated inflammatory responses. J. Biol. Chem. 287, 11579–11591. doi: 10.1074/jbc.M111.336032
Richards, J. G. (2011). Physiological, behavioral and biochemical adaptations of intertidal fishes to hypoxia. J. Exp. Biol. 214(Pt. 2), 191–199. doi: 10.1242/jeb.047951
Seaman, M. N. L. (1991). Survival and aspects of metabolism in oysters, Crassostrea gigas, during and after prolonged air storage. Aquaculture 93, 389–395. doi: 10.1016/0044-8486(91)90228-Y
Sokolova, I. M., and Pörtner, H. O. (2001). Physiological adaptations to high intertidal lifeinvolve improved water conservation abilities and metabolic rate depression in Littorina saxatilis. Mar. Ecol. Prog. Ser. 224, 171–186. doi: 10.3354/meps224171
Strachan, S. R., Chester, E. T., and Robson, B. J. (2015). Freshwater invertebrate life history strategies for surviving desiccation. Springer Sci. Rev. 3, 57–75. doi: 10.1007/s40362-015-0031-9
Su, W., Rong, J., Zha, S., Yan, M., Fang, J., and Liu, G. (2018). Ocean acidification affects the cytoskeleton, lysozymes, and nitric oxide of hemocytes: a possible explanation for the hampered phagocytosis in blood clams, Tegillarca granosa. Front. Physiol. 9:619. doi: 10.3389/fphys.2018.00619
Sun, Y., Zhou, Z., Wang, L., Yang, C., Jianga, S., and Song, L. (2014). The immunomodulation of a novel tumor necrosis factor (CgTNF-1) in oyster Crassostrea gigas. Dev. Comp. Immunol. 45, 291–299. doi: 10.1016/j.dci.2014.03.007
Teixeira, T., Diniz, M., Calado, R., and Rosa, R. (2013). Coral physiological adaptations to air exposure: Heat shock and oxidative stress responses in Veretillum cynomorium. J. Exp. Mar. Biol. Ecol. 439, 35–41. doi: 10.1016/j.jembe.2012.10.010
Tripathi, P., Tripathi, P., Kashyap, L., and Singh, V. (2007). The role of nitric oxide in inflammatory reactions. FEMS Immunol. Med. Microbiol. 51, 443–452. doi: 10.1111/j.1574-695X.2007.00329.x
Vetter, S. W., and Leclerc, E. (2003). Novel aspects of calmodulin target recognition and activation. Eur. J. Biochem. 270, 404–414. doi: 10.1046/j.1432-1033.2003.03414.x
Wang, T., Meng, J., Li, L., and Zhang, G. (2016). Characterization of CgHIFα-Like, a novel bHLH-PAS Transcription Factor Family Member, and its role under hypoxia stress in the Pacific oyster Crassostrea gigas. PLoS One 11:e0166057. doi: 10.1371/journal.pone.0166057
Wang, W., Li, M., Wang, L., Chen, H., Liu, Z., Jia, Z., et al. (2017). The granulocytes are the main immunocompetent hemocytes in Crassostrea gigas. Dev. Comp. Immunol. 67, 221–228. doi: 10.1016/j.dci.2016.09.017
Xin, L., Zhang, H., Du, X., Li, Y., Li, M., Wang, L., et al. (2016). The systematic regulation of oyster CgIL17-1 and CgIL17-5 in response to air exposure. Dev. Comp. Immunol. 63, 144–155. doi: 10.1016/j.dci.2016.06.001
Xu, B., Chen, S., Luo, Y., Chen, Z., Liu, L., Zhou, H., et al. (2011). Calcium signaling is involved in cadmium-induced neuronal apoptosis via induction of reactive oxygen species and activation of MAPK/mTOR network. PLoS One 6:e19052. doi: 10.1371/journal.pone.0019052
Xu, J., Jiang, S., Li, Y., Li, M., Cheng, Q., Zhao, D., et al. (2016). Caspase-3 serves as an intracellular immune receptor specific for lipopolysaccharide in oyster Crassostrea gigas. Dev. Comp. Immunol. 61, 1–12. doi: 10.1016/j.dci.2016.03.015
Yamada, K., Nakata, M., Horimoto, N., Saito, M., Matsuoka, H., and Inagaki, N. (2000). Measurement of glucose uptake and intracellular calcium concentration in single, living pancreatic β-cells. J. Biol. Chem. 275, 22278–22283. doi: 10.1074/jbc.M908048199
Zhang, G., Fang, X., Guo, X., Li, L., Luo, R., Xu, F., et al. (2012). The oyster genome reveals stress adaptation and complexity of shell formation. Nature 490, 49–54. doi: 10.1038/nature11413
Zhang, G., Li, L., Meng, J., Qi, H., Qu, T., Xu, F., et al. (2016). Molecular Basis for Adaptation of Oysters to Stressful Marine Intertidal Environments. Annu. Rev. Anim. Biosci. 4, 357–381. doi: 10.1146/annurev-animal-022114-110903
Zhang, Y., Sun, J., Mu, H., Li, J., Zhang, Y., Xu, F., et al. (2015). Proteomic basis of stress responses in the gills of the pacific oyster Crassostrea gigas. J. Prot. Res. 14, 304–317. doi: 10.1021/pr500940s
Zheng, Y., Liu, Z., Wang, L., Li, M., Zhang, Y., Zong, Y., et al. (2020). A novel tumor necrosis factor in the Pacific oyster Crassostrea gigas mediates the antibacterial response by triggering the synthesis of lysozyme and nitric oxide. Fish Shellfish Immunol. 98, 334–341. doi: 10.1016/j.fsi.2019.12.073
Keywords: intertidal zone, calmodulin, nitrite oxide, tumor necrosis factor, aerial exposure
Citation: Chen H, Xin L, Wang L, Zhang H, Liu R, Wang H, Qiao X, Wang L and Song L (2021) Ca2 +/Calmodulin-NOS/NO-TNFs Pathway Hallmarks the Inflammation Response of Oyster During Aerial Exposure. Front. Mar. Sci. 7:603825. doi: 10.3389/fmars.2020.603825
Received: 08 September 2020; Accepted: 30 November 2020;
Published: 11 January 2021.
Edited by:
Valerio Matozzo, University of Padua, ItalyReviewed by:
Loriano Ballarin, University of Padua, ItalyUmberto Rosani, Alfred Wegener Institute Helmholtz Centre for Polar and Marine Research (AWI), Germany
Guangxu Liu, Zhejiang University, China
Copyright © 2021 Chen, Xin, Wang, Zhang, Liu, Wang, Qiao, Wang and Song. This is an open-access article distributed under the terms of the Creative Commons Attribution License (CC BY). The use, distribution or reproduction in other forums is permitted, provided the original author(s) and the copyright owner(s) are credited and that the original publication in this journal is cited, in accordance with accepted academic practice. No use, distribution or reproduction is permitted which does not comply with these terms.
*Correspondence: Huan Zhang, zhanghuan@qdio.ac.cn; Lingling Wang, wanglingling@dlou.edu.cn