Abstract
We report on the effects of 3.5 MeV proton irradiation on single crystals of the magnetic superconductor EuFe2(As1 − xPx)2, investigated at microwave frequencies by a coplanar waveguide resonator technique. We studied the relative strength of the two collective phenomena by analyzing the dependence of their onset temperatures on different perturbations: magnetic fields and structural disorder. Results suggest that superconductivity and magnetism in this material are two competing orders: as the former is suppressed by irradiation or by excess P doping, the latter reinforces and manifests itself at higher temperatures. The ability to modulate superconductivity and magnetism in EuFe2(As1 − xPx)2 by disorder and in-plane magnetic field, respectively, offers the chance to tailor, even on a local scale, their relative weights. This could be useful for the aim of engineering this material for specific applications.
Export citation and abstract BibTeX RIS
1. Introduction
Interplay of magnetism and superconductivity is an open and intriguing topic in contemporary condensed matter research. Although these two phenomena are traditionally antagonistic, there is evidence that unconventional superconductivity is closely linked to magnetism. An example is given by iron-based superconductors (IBSs), where the coupling mechanism at the origin of superconductivity involves antiferromagnetic spin fluctuations [1]. IBS systems containing magnetic rare-earth-metal elements are of even further interest, since they also show magnetic ordering of local moments. This is the case, for example, of the EuFe2As2 parent compound, with the Eu2 + moments aligned along the a-axis direction and ordered antiferromagnetically along the c-axis direction [2]. Superconductivity arises in this system if chemical pressure is applied through the isovalent substitution of P in the site of As [3]. In the resulting EuFe2(As1 − xPx)2 compound, it was found that Eu2 + magnetic moments cant out of the ab plane, yielding a net ferromagnetic component along the c-axis direction. This magnetic superconductor has already been studied [4–9], but its rich phenomenology is far from being fully addressed, to date.
Besides the great interest to understand the fundamental mechanism of interplay between superconductivity and magnetism, mastering magnetic and superconducting properties of such materials could open to new spintronics applications exploiting e.g. Higgs modes [10] or spin Hall effects [11]. An approach useful both for the fundamental study and the engineering of materials makes use of the controlled introduction of disorder.
The role of disorder in superconductors is twofold. On the one hand, nanoscale defects act as scattering centers for charge carriers, yielding critical temperature and superfluid fraction suppression [12, 13]. On the other hand, they improve pinning of fluxons, resulting in a lower dissipation for a superconductor in the presence of a magnetic field [14, 15]. Moreover, when also a long-range magnetic order sets in, e.g. with the formation of magnetic domains, one should consider that disorder could also affect the dynamics of domain walls [16, 17]. Thus, disorder on a magnetic superconductor can lead to complex phenomenology, and the investigation of the effects of disorder in the new generations of magnetic superconductors is just at the beginnings [18]. One of the most efficient methods to induce controlled disorder into materials, without contributing extra charge doping and huge structural modifications, is ion irradiation.
In this paper, we report on the effect of 3.5 MeV proton irradiation of EuFe2(As1 − xPx)2 single crystals. To characterize the samples, we used a coplanar waveguide resonator (CPWR) technique, which was first described in references [19, 20]. This technique was used to study different families and several compounds of IBSs, even before and after the introduction of disorder by ion irradiation, giving hints towards the comprehensive understanding of the order parameter symmetry in these materials [12, 13, 21–24]. Here we show that CPWR is a sensitive probe suitable not only to detect the diamagnetic signature of superconductivity, but also to reveal a bulk magnetic response, making this technique particularly reliable for the study of samples where both are present, as in the case of magnetic superconductors.
We analyzed two kinds of samples with two doping levels, one close to optimal doping and another one with a small excess of P, resulting in a slightly lower superconducting critical temperature. Thus, we could also compare the effects of two kinds of disorder, of chemical origin and induced by irradiation. In this work, we focus on the onset temperatures of both the superconducting and the magnetic responses, to understand whether and how they are influenced by disorder and/or applied magnetic field.
The paper is organized as follows. Experimental details are given in section 2, while section 3 describes in brief the CPWR technique. In section 4, we report on results obtained on a pristine sample with the optimal x = 0.20 doping, while section 5 describes the effects of proton irradiation on its properties. In section 6, we analyze the effects of irradiation on a crystal with x = 0.23 and lower pristine superconducting critical temperature. Finally, the whole dataset is discussed in section 7, where also conclusions are drawn. In the appendix, the CPWR method is described with further details, showing how the data analysis can be modified with respect to the case of a pure superconductor to take into account the bulk magnetic contribution.
2. Experimental details
Single crystals of EuFe2(As1 − xPx)2 were grown by a self-flux method [25, 26]. Crystals with different P doping levels, x = 0.20 and 0.23, were grown using different precursors (Eu, Fe, P, As and Eu, FeAs, FeP, respectively), and show different superconducting critical temperatures, . Crystals with x = 0.20 are close to the optimal doping and show
K, while crystals with a small excess of P (x = 0.23) show a suppression of
of about 2 K, due to the very narrow superconducting dome in the phase diagram [7]. For the sake of comparison, we also measured a EuFe2As2 crystal (with magnetic but not superconducting behavior) and a BaFe2(As1 − xPx)2 crystal (superconducting but not magnetic). The BaFe2(As1 − xPx)2 crystal was already analyzed and discussed in reference [9], but here in addition we report data about its
after proton irradiation.
The investigated samples were cleaved and shaped in the form of thin plates, whose thickness, in the range of 10 µm, was at least ten times smaller than width and length.
Samples under study were mounted on top of the central stripline of a coplanar waveguide resonator for microwave characterization [19]. The CPWR consists of a patterned YBa2Cu3O7 − x film grown on an MgO substrate (details in [27]). Resonance frequency f and unloaded quality factor Q were measured by a vector network analyzer, as a function of temperature. Dc magnetic field was applied parallel to the resonator plane, i.e. parallel to the crystal ab-planes. Data were processed according to the analysis approach described in the next section and in the
Proton irradiations were performed at the CN facility of the LNL laboratories of the Italian National Institute for Nuclear Physics (INFN). The samples were irradiated with 3.5-MeV protons in multiple sessions, up to total fluences of the order of 1017 cm−2. By means of Monte Carlo simulations performed with the PHITS [28] and SRIM [29] codes, the implantation depth was estimated to be of about 67 µm, a value exceeding the crystal thickness by enough to avoid implantation and to assure a quite uniform defect density. We also calculated the dpa (displacements per atom), as a measure of the damage introduced into the material by irradiation, and found a dpa-to-fluence ratio of about 3 × 10−20 cm2.
3. CPWR technique and data analysis
The coplanar-waveguide-resonator technique allows determining the complex permeability of a small sample coupled to the resonator, from the changes it induces to the resonance frequency and quality factor, within a cavity perturbation approach. The method is fully described in the appendix. Here we report the formulae useful to derive the physical quantities that are discussed in this work, from the analysis of experimental data.
We consider that the complex magnetic susceptibility of the sample under investigation is made of two parts: one stems from the response of the bulk to an applied field, , while the other one originates from the screening effect,
, where
, λL is the London penetration depth, and σn is the quasiparticle conductivity.
It turns out (see appendix) that:


where Δf/f and Δ(1/Q) are the experimental shifts of the resonance frequency and of the inverse of the quality factor induced by the presence of the sample under test, and Γf and ΓQ are geometrical factors that can be determined by a self-consistent procedure, which takes into account also the finite size of the crystal and the consequent demagnetization effects [19].
Figure 1 shows what happens to the resonance frequency of the resonator when the crystal coupled to it undergoes a superconducting or a magnetic transition, or both. From equation (1) it turns out that a change in the real part of the susceptibility produces an opposite change in the resonance frequency: . Thus, when upon cooling the superconducting transition occurs and the London penetration is drastically reduced (strong diamagnetic behavior, with an increasingly negative
), the resonance frequency increases. This is shown in figure 1 for a superconducting BaFe2(As1 − xPx)2 crystal (blue arrow). On the other hand, when a magnetic transition occurs with increasing positive
, the resonance frequency decreases, as shown in figure 1 for a EuFe2As2 crystal (red arrow). The EuFe2(As1 − xPx)2 crystals display both transitions.
Figure 1. Resonance frequency of the resonator coupled to a superconducting BaFe2(As1 − xPx)2 crystal (SC), to a magnetic EuFe2As2 crystal (M), and to the magnetic superconductor EuFe2(As1 − xPx)2 (SC+M). The curves are vertically shifted, for the sake of clarity. The onset of a strong diamagnetic signal causes an increase of the resonance frequency (blue arrows), while the onset of a bulk positive magnetic susceptibility causes a drop of the resonance frequency (red arrows). The dashed line in the lowest curve is a qualitative continuation of the purely superconductive behavior towards lower temperatures, to highlight the downward contribution of the positive bulk susceptibility.
Download figure:
Standard image High-resolution imageOnce the second members of equations (1) and (2) are determined as a function of temperature, from the experimental data and the calibration procedure, one can try to distinguish between the screening effect of supercurrents and the bulk susceptibility , for samples where superconductivity and magnetism coexist. In the range between the superconducting transition temperature,
, and the onset of the magnetic signal,
, we assume
= 0 and extract λL(T) and σn(T) from
and
(see figure 2). Then, we extrapolate these curves to lower temperatures (note that below
they are quite flat) and subtract them to the
and
low-temperature data. Thus finally the real and imaginary parts of
can be calculated below
from equations (1) and (2) with
(figure 2).
Figure 2. Data analysis. (a) data from the experiment, after calibration. The temperature range is divided into three parts: above the crystal is in the normal state, in between
and
a pure superconducting state is developed, while below
also a magnetic signal emerges. Above
the standard analysis [19] can be done, by equations (1) and (2) with
= 0, yielding the London penetration depth and the quasiparticle conductivity ((b), right scales). Data of panel (a) in the superconducting range, are extrapolated to lower temperatures and subtracted to data in the SC+M state. Thus, complex
can be extracted by equations (1) and (2) with
((b), left scale).
Download figure:
Standard image High-resolution image4. Pristine x = 0.20 sample
Figure 2 shows that the normal to superconducting phase transition is qualitatively similar to non-magnetic superconductors of the same family, e.g. BaFe2(As1 − xPx)2 [9], with slightly larger absolute values of the London penetration depth. Below , positive bulk susceptibility emerges, with a two-step increase of the real part
, associated to two broad peaks of the imaginary part
. This detailed analysis confirms the model described in the previous section and the picture that can be found in literature for this compound. On the other hand, due to the fact that in the low-temperature range the screening term is quite flat, the features in
we are interested in discussing in this paper can easily be recognized directly looking at raw data. In particular, the onset of the
signal is clearly visible in 1/Q(T) measurements of the resonator with the coupled crystal. This is why hereafter we directly show, compare, and discuss raw data from the experiment.
Figure 3 shows the effects of the application of a dc field parallel to ab-planes of the crystal, for a pristine sample with x = 0.20. As the dc field is increased, first the 1/Q highest peak, associated to a corresponding peak, shifts slightly towards higher temperatures. Then, after merging with the smaller peak at higher temperature, it shifts towards lower temperatures, until it completely disappears from the observation window when the dc field approaches 0.5 T. The nature of these peaks is related to the complex interplay of the two orders through the spontaneous appearance of vortices and to some dynamics, as already discussed elsewhere [9], and is out of the scope of the present paper. On the contrary, the superconducting
is much less affected by the application of the dc field, indicating that the applied fields are significantly lower than the upper critical field Bc2.
5. Effects of proton irradiation
Irradiations with 3.5 MeV protons lead to the formation of nano-size defects and small clusters and cascades, quite uniformly distributed into the crystal volume. As already pointed out in the Introduction, these defects are potentially active as scattering centers for charge carriers as well as pinning centers, for both fluxons and domain walls, influencing their dynamics. Figure 4 shows the effects of proton irradiation on 1/Q for the same crystal of figure 3, for two different fluences, 4.7 × 1016 and 9.5 × 1016 cm−2.
Figure 3. Inverse of the quality factor of the resonator with the pristine sample coupled to it. Raw data are shown as a function of temperature, for different values of dc field, applied parallel to the crystal ab-planes.
Download figure:
Standard image High-resolution imageFigure 4. Same graphs as in figure 3, but after irradiation of the same crystal up to fluences 4.7 × 1016 cm−2 (a) and 9.5 × 1016 cm−2 (b).
Download figure:
Standard image High-resolution imageIt can be noticed that superconductivity is suppressed by irradiation ( is downshifted), while the bulk magnetic signal shows an opposite trend. This is shown more clearly in figure 5, where we summarize the behaviors of the reference temperatures, as a function of dc magnetic field and for the above mentioned proton irradiation fluences.
is slightly influenced by an in-plane dc field, while it is clearly suppressed by irradiation. On the contrary,
is suppressed appreciably by dc field dependence, while it is slightly improved by irradiation. The effect of irradiation, extended to a greater number of fluences, is reported in the inset, where the opposite trends of
and
as a function of irradiation dose are confirmed.
suppression for the purely superconducting BaFe2(As1 − xPx)2 is also reported, as a comparison. The superconducting transitions for the two compounds show similar behaviors.
Figure 5. Dependence of and
of a x = 0.20 sample, on in-plane magnetic field and irradiation fluence. In the main panel, data are shown as a function of dc field, for the pristine crystal and for two reference proton doses (irrad. II corresponds to a fluence of 4.7 × 1016 cm−2 and irrad. IV to 9.5 × 1016 cm−2). Gray arrows mark the opposite effect of irradiation on the two reference temperatures. This is more evident in the inset, where
and
are shown to converge at increasing the irradiation fluence. The suppression of the critical temperature of a superconducting BaFe2(As1 − xPx)2 sample is also shown, for a comparison.
Download figure:
Standard image High-resolution image6. Analysis of a sample with x = 0.23
Measurements on the sample with P doping x = 0.23 are reported in figure 6. The pristine crystal shows a behavior which is very similar to that of the crystal with x = 0.20 after irradiation at the highest dose. In particular, it shows close to
. On the other hand, after irradiation
decreases below
and the superconducting transition is completely hidden by the bulk magnetic contribution (figure 6(b)). The only way to reveal the superconducting transition is to apply a magnetic field, shifting the bulk
out of the observed temperature range. For the curves in (b), this occurs for
= 1 T (red curve), with a decrease of dissipation, hallmark of the superconducting transition, below about 11 K. As for the optimally doped crystal, the sample with x = 0.23 shows that the suppression of
with field is less pronounced after irradiation (panel (c)).
Figure 6. Raw 1/Q data for a sample with x = 0.23, as a function of temperature and for different values of in-plane applied dc magnetic field. Pristine sample is shown in (a) and the same sample irradiated up to Φ = 9.0 × 1016 cm−2, in (b). The onset temperature of the bulk magnetic signal is reported as a function of field in (c), showing that is enhanced by irradiation.
Download figure:
Standard image High-resolution image7. Discussion and conclusions
The key results of this study are summarized in figure 7. With the aim of comparing the effects of irradiation on the superconducting and magnetic components, we show the onset temperatures of both, i.e. the superconducting transition and the onset temperature of the bulk
,
, as a function of proton fluence. Moreover, with the aim of comparing the effects of disorder of chemical origin, due to excess P doping, with disorder induced by irradiation, we show data from the samples with x = 0.20 (panel (a)) and with x = 0.23 (panel (b)), with the same temperature scales. To connect the two sections of figure 7, we exploit the fact that the pristine x = 0.23 sample looks very similar to the x = 0.20 sample irradiated at the highest dose (see above). Thus we simply merged the graphs, keeping the same scale increments on both abscissae, in such a way to connect the curves on both sides. The idea is that if the curves cross the two parts of the graph with the same trend, this can be interpreted as a strong indication that the two sources of disorder are additive and in fact they affect the reference temperatures in a similar way. The result is quite impressive:
is clearly continuous in its linear increase, and also
shows the same trend in (a) and (b). Concerning
, we were forced to show values from measurements with different applied fields, because for the x = 0.23 sample it was possible to identify the superconducting transition only in the presence of a dc field of 0.5 − 1 T, while with the x = 0.20 crystal we applied 0.5 T as a maximum. Nevertheless, the variability of
with dc field is small enough (we reported both 0, 0.5, and 1 T data) to let us state that the same decreasing trend is shown by
across the two sections of the graph. We avoided to report
values too close to
, since the definition of the critical temperature can be problematic, in these cases (most irradiated x = 0.20, and pristine x = 0.23).
Figure 7. and
as a function of disorder. The two sections of the graph report results for crystals with dopings x = 0.20 (a) and x = 0.23 (b). Vertical scales (temperatures) are identical. Horizontal scales represent irradiation fluence (same scale increments for both (a) and (b)). The two sections were merged in such a way to give continuity to the curves (see text).
and
values were collected with the applied field reported in the apex (see legend).
Download figure:
Standard image High-resolution imageFigure 7 seems to suggest that superconductivity and magnetism in EuFe2(As1 − xPx)2 are two quite independent but competing orders: as the former is suppressed by irradiation or by excess P doping, the latter is reinforced and manifests itself at higher temperatures. Moreover, possible generation of magnetic impurities by proton irradiation should be also considered, as already observed in P-doped [26] and Rh-doped [31] Ba122 compounds. Thus, we observe in figure 7 the transition from a ferromagnetic superconductor (with ) to a superconducting ferromagnet (with
), as reported for RbEu(Fe1 − xNix)4As4 as a function of Ni doping [30]. Of course, this is not the full story. Here we focused our study only onto the analysis of the onset temperatures of the superconductive and magnetic components. A comprehensive understanding of the way how they interplay when coexisting is still to be achieved, and we are confident it will spark numerous new dedicated experiments. Anyway, the CPWR technique described here showed to be potentially a useful tool for the study of magnetic superconductors, including the microwave range in the spectrum of possible characterizations [9].
From the practical point of view, we have shown that superconductivity and magnetism can be modulated by disorder and magnetic field, respectively, offering the chance to tailor (even on a local scale) their relative weights. This could be useful for the aim of engineering such materials for specific applications.
Acknowledgments
This work was partially supported by the Italian Ministry of Education, University and Research (Project PRIN HIBiSCUS, Grant No. 201785KWLE), by a Grant-in-Aid for Scientific Research (A) (17H01141) from the Japan Society for the Promotion of Science (JSPS), and by the National Key Research and Development Program of China (2016YFA0300202). Irradiations were performed within the framework of the INFN-Politecnico di Torino M.E.S.H. Research Agreement.
Appendix.: Further details on the CPWR technique
The resonance frequency and the quality factor of a resonator can be expressed through the complex angular frequency , as
and
, respectively [32]. These quantities change when the sample to be investigated is coupled to the resonator, and, from these changes, the complex permittivity and complex permeability of the sample can be determined. When the sample under study is much smaller than the effective volume of the cavity, a cavity-perturbation approach can be adopted. A requirement is that the crystal does not change significantly the external distribution of electromagnetic fields. In such conditions, the angular frequency shift due to the crystal can be expressed by [32]

where the index 0 is for the resonator without the sample. Complex conjugates are indicated by asterisks, is the sample volume, and
is the volume of the effective cavity. In our experiment, the crystal is mounted in a region of the resonator where electric fields are negligible. If, in addition, the perturbation to the magnetic field can be considered small (
), and assuming that
and
, the equation above gives:

The second member of this equation can be rewritten in terms of complex magnetic susceptibility χ as , where
resumes the geometrical factors and
is the sum of the bulk magnetic response and the effect of the overall screening, respectively. Concerning the screening by supercurrents, in the case of an infinite slab of half-thickness c, when the rf magnetic field is applied parallel to its face, if losses are negligible and the low-temperature limit is considered, then
and
, while in the case losses can not be neglected [33, 34]:

where . Thus, from the experimental shifts of the resonance frequency and of the quality factor inverse, the following quantities (corresponding to equations (1) and (2) in the main text) can be calculated:
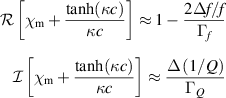
Actually, two different geometrical factors are considered here, Γf and ΓQ, since the adopted approximations affect in a slightly different way the imaginary and real parts of equation (A1) [32]. They can be determined from data above , where we assume the sample behaves like a metal, with
, inverse of the classical skin depth
, and with
= 0. These assumptions, in combination with equations (1) and (2), allows one to fit Δf/f and Δ(1/Q) data above
, obtaining Γf and ΓQ. We discussed elsewhere [19] the full calibration technique, taking also into account the finite dimensions of the sample and the resultant demagnetization effect.